Hydrogel 3d printer
3D printing of hydrogel composite systems: Recent advances in technology for tissue engineering
1. Wang X, Jiang M, Zhou Z W, et al. 3D printing of polymer matrix composites:A review and prospective. Compos B Eng. 2017;110:442–458. http://dx.doi.org/10.1016/j.compositesb.2016.11.034. [Google Scholar]
2. Chua C K, Leong K F. 3D printing and additive manufacturing :Principles and applications. 4th ed. Singapore: World Scientific Publishing; 2015. [Google Scholar]
3. Billiet T, Vandenhaute M, Schelfhout J, et al. A review of trends and limitations in hydrogel-rapid prototyping for tissue engineering. Biomaterials. 2012;33(26):6020–6041. http://dx.doi.org/10.1016/j.biomaterials.2012.04.050. [PubMed] [Google Scholar]
4. Ballyns J J, Gleghorn J P, Niebrzydowski V, et al. Image-guided tissue engineering of anatomically shaped implants via MRI and micro-CT using injection molding. Tissue Eng Part A. 2008;14(7):1195–1202. http://dx.doi.org/10.1089/ten.tea.2007.0186. [PubMed] [Google Scholar]
5. Chia H N, Wu B M. Recent advances in 3D printing of biomaterials. J Biol Eng. 2015;9(1):4. http://dx.doi.org/10.1186/S13036-015-0001-4. [PMC free article] [PubMed] [Google Scholar]
6. Seyednejad H, Gawlitta D, Kuiper R V, et al. In vivo biocompatibility and biodegradation of 3D-printed porous scaffolds based on a hydroxyl-functionalized poly(epsiloncaprolactone) Biomaterials. 2012;33(17):4309–4318. http://dx.doi.org/10.1016/j.biomaterials.2012.03.002. [PubMed] [Google Scholar]
7. Wu G H, Hsu S H. Review:Polymeric-Based 3D printing for tissue engineering. J Med Bioeng. 2015;35(3):285292. http://dx.doi.org/10.1007/s40846-015-0038-3. [PMC free article] [PubMed] [Google Scholar]
8. Utech S, Boccaccini A R. A review of hydrogelbased composites for biomedical applications:Enhancement of hydrogel properties by addition of rigid inorganic fillers. J Mater Sci. 2016;51(1):271–310. http://dx.doi.org/10.1007/s10853-015-9382-5. [Google Scholar]
9. Gaharwar A K, Peppas N A, Khademhosseini A. Nanocomposite hydrogels for biomedical applications. Biotechnol Bioeng. 2014;111(3):441–453. http://dx.doi.org/10.1002/bit.25160. [PMC free article] [PubMed] [Google Scholar]
10. Xu K, Wang J H, Chen Q, et al. Spontaneous volume transition of polyampholyte nanocomposite hydrogels based on pure electrostatic interaction. J Colloid Interface Sci. 2008;321(2):272–278. http://dx.doi.org/10.1016/jjcis.2008.02.024. [PubMed] [Google Scholar]
11. Kabiri K, Omidian H, Zohuriaan-Mehr M J, et al. Superabsorbent hydrogel composites and nanocomposites:A review. Polym Compos. 2011;32(2):277–289. http://dx.doi.org/10.1002/pc.21046. [Google Scholar]
12. Thoniyot P, Tan M J, Karim A A, et al. NanoparticleHydrogel composites:Concept, design, and applications of these promising, multi-functional materials. Adv Sci. 2015;2(1-2) http://dx.doi.org/10.1002/Advs.201400010. [PMC free article] [PubMed] [Google Scholar]
13. Lee J W, Kim S Y, Kim S S, et al. Synthesis and characteristics of interpenetrating polymer network hydrogel composed of chitosan and poly(acrylic acid) J Appl Polym Sci. 1999;73(1):113–120. http://dx.doi.org/10.1002/(SICI)1097-4628(19990705)73:1<113::AID-APP13>3.0.C0;2-D. [Google Scholar]
14. Ehrburger P, Donnet J B. Interface in compositematerials. Philos Trans A Math Phys Eng Sci. 1980;294(1411):495–505. http://dx.doi.org/10.1098/rsta.1980.0059. [Google Scholar]
15. Jeong S H, Koh Y H, Kim S W, et al. Strong and biostable hyaluronic acid-calcium phosphate nanocomposite hydrogel via in situ precipitation process. Biomacromolecules. 2016;17(3):841–851. http://dx.doi.org/10.1021/acs.biomac.5b01557. [PubMed] [Google Scholar]
16. Wust S, Godla M E, Muller R, et al. Tunable hydrogel composite with two-step processing in combination with innovative hardware upgrade for cell-based three-dimensional bioprinting. Acta Biomater. 2014;10(2):630–640. http://dx.doi.org/10.1016/j.actbio.2013.10.016. [PubMed] [Google Scholar]
17. Duan B, Hockaday L A, Kang K H, et al. 3D Bioprinting of heterogeneous aortic valve conduits with alginate/gelatin hydrogels. J Biomed Mater Res A. 2013;101(5):1255–1264. http://dx.doi.org/10.1002/jbm.a.34420. [PMC free article] [PubMed] [Google Scholar]
18. Melchels F P W, Feijen J, Grijpma D W. A review on stereolithography and its applications in biomedical engineering. Biomaterials. 2010;31(24):6121–6130. http://dx.doi.org/10.1016/j.biomaterials.2010.04.050. [PubMed] [Google Scholar]
19. Bertsch A, Jiguet S, Bernhard P, et al. Microstereolithography A review. Rapid Prototyping Technologies. 2003;758:3–15. [Google Scholar]
20. Beluze L, Bertsch A, Renaud P. Microstereolithography:A new process to build complex 3D objects Design. Test, and Microfabrication of Mems and Moems, Pts 1 and. 1999;2;3680:808–817. http://dx.doi.org/10.1117/12.341277. [Google Scholar]
21. Choi J S, Kang H W, Lee I H, et al. Development of micro-stereolithography technology using a UV lamp and optical fiber. Int J Adv Manuf Technol. 2009;41(3-4):281–286. http://dx.doi.org/10.1007/s00170-008-1461-1. [Google Scholar]
22. Bertsch A, Renaud P, Vogt C, et al. Rapid prototyping of small size objects. Rapid Prototyp J. 2000;6(4):259–266. http://dx.doi.org/10.1108/13552540010373362. [Google Scholar]
23. Sun C, Fang N, Wu D M, et al. Projection microstereolithography using digital micro-mirror dynamic mask. Sens Actuators A Phys. 2005;121(1):113–120. http://dx.doi.org/10.1016/j.sna.2004.12.011. [Google Scholar]
24. Ambrosio L. Biomedical composites. 2nd ed. UK: Woodhead Publishing; 2010. [Google Scholar]
25. Maruo Sand Ikuta K. Submicron stereolithography for the production of freely movable mechanisms by using singlephoton polymerization. Sens Actuators A Phys. 2002;100(1):70–76. http://dx.doi.org/10.1016/S0924-4247(02)00043-2. [Google Scholar]
26. Lee K S, Kim R H, Yang D Y, et al. Advances in 3D nano/microfabrication using two-photon initiated polymerization. Prog Polym Sci. 2008;33(6):631–681. http://dx.doi.org/10.1016/j.progpolymsci.2008.01.001. [Google Scholar]
27. Weiss T, Hildebrand G, Schade R, et al. Two-Photon polymerization for microfabrication of three-dimensional scaffolds for tissue engineering application. Eng Life Sci. 2009;9(5):384–390. http://dx.doi.org/10.1002/elsc.200900002. [Google Scholar]
28. Ostendorf A, Chichkov B N. Two-photon polymerization:A new approach to micromachining. Photonics Spectra. 2006;40(10):72–80. [Google Scholar]
29. Hutmacher D W, Sittinger M, Risbud M V. Scaffold-based tissue engineering:Rationale for computeraided design and solid free-form fabrication systems. Trends Biotechnol. 2004;22(7):354–362. http://dx.doi.org/10.1016/j.tibtech.2004.05.006. [PubMed] [Google Scholar]
30. Bikas H, Stavropoulos P, Chryssolouris G. Additive manufacturing methods and modelling approaches:A critical review. Int J Adv Manuf Technol. 2016;83(1-4):389–405. http://dx.doi.org/10.1007/s00170-015-7576-2. [Google Scholar]
31. Anitha R, Arunachalam S, Radhakrishnan P. Critical parameters influencing the quality of prototypes in fused deposition modelling. J Mater Process Technol. 2001;118(1):385–388. http://dx.doi.org/10.1016/S0924-0136(01)00980-3. [Google Scholar]
32. Xiong Z, Yan Y, Zhang R, et al. Fabrication of porous poly (L-lactic acid) scaffolds for bone tissue engineering via precise extrusion. Scr Mater. 2001;45(7):773–779. http://dx.doi.org/10.1016/S1359-6462(01)01094-6. [Google Scholar]
33. Greulich M, Greul M, Pintat T. Fast, functional prototypes via multiphase jet solidification. Rapid Prototyp J. 1995;1(1):20–25. http://dx.doi.org/10.1108/13552549510146649. [Google Scholar]
34. Shor L, Güçeri S, Chang R, et al. Precision extruding deposition (PED) fabrication of polycaprolactone (PCL) scaffolds for bone tissue engineering. Biofabrication. 2009;1(1):015003. http://dx.doi.org/10.1088/1758-5082/1Z1/015003. [PubMed] [Google Scholar]
35. Torres J, Cotelo J, Karl J, et al. Mechanical property optimization of FDM PLA in shear with multiple objectives. JOM. 2015;67(5):1183–1193. http://dx.doi.org/10.1007/s11837-015-1367-y. [Google Scholar]
36. Yeong W Y, Chua C K, Leong K F, et al. Rapid prototyping in tissue engineering:Challenges and potential. Trends Biotechnol. 2004;22(12):643–652. http://dx.doi.org/10.1016/j.tibtech.2004.10.004. [PubMed] [Google Scholar]
37. Gates R D, Baghdasarian G, Muscatine L. Temperature stress causes host-cell detachment in symbiotic cnidarians-implications for coral bleaching. Biol Bull. 1992;182(3):324–332. http://dx.doi.org/10.2307/1542252. [PubMed] [Google Scholar]
38. Landers R, Mülhaupt R. Desktop manufacturing of complex objects, prototypes and biomedical scaffolds by means of computer-assisted design combined with computer-guided 3D plotting of polymers and reactive oligomers. Macromol Mater Eng. 2000;282(1):17–21. http://dx.doi.org/10.1002/1439-2054(20001001)282:1<17::AIDMAME17>3.0.CO;2-8. [Google Scholar]
39. Billiet T, Gevaert E, De Schryver T, et al. The 3D printing of gelatin methacrylamide cell-laden tissue-engineered constructs with high cell viability. Biomaterials. 2014;35(1):49–62. http://dx.doi.org/10.1016/j.biomaterials.2013.09.078. [PubMed] [Google Scholar]
40. Luo Y, Lode A, Akkineni A R, et al. Concentrated gelatin/alginate composites for fabrication of predesigned scaffolds with a favorable cell response by 3D plotting. RSC Adv. 2015;5(54):43480–43488. http://dx.doi.org/10.1039/C5RA04308E. [Google Scholar]
41. Akkineni A R, Luo Y, Schumacher M, et al. 3D plotting of growth factor loaded calcium phosphate cement scaffolds. Acta Biomater. 2015;27:264–274. http://dx.doi.org/10.1016/j.actbio.2015.08.036. [PubMed] [Google Scholar]
42. Yilgor P, Sousa R A, Reis R L, et al. 3D plotted PCL scaffolds for stem cell based bone tissue engineering, Macromol Symp, 2008. Wiley Online Library. 269:92–99. http://dx.doi.org/10.1002/masy.200850911. [Google Scholar]
43. Landers R, Mulhaupt R. Desktop manufacturing of complex objects, prototypes and biomedical scaffolds by means of computer-assisted design combined with computer-guided 3D plotting of polymers and reactive oligomers. Macromol Mater Eng. 2000;282(9):17–21. http://dx.doi.org/10.1002/1439-2054(20001001)282:1<17::AidMame17>3.0.Co;2-8. [Google Scholar]
44. Smay J E, Gratson G M, Shepherd R F, et al. Directed colloidal assembly of 3D periodic structures. Adv Mater. 2002;14(18):1279–1283. [Google Scholar]
45. Ahn B Y, Duoss E B, Motala M J, et al. Omnidirectional printing of flexible, stretchable, and spanning silver microelectrodes. Science. 2009;323(5921):1590–1593. https:// dx.doi.org/10.1126/science.1168375. [PubMed] [Google Scholar]
46. Vozzi G, Previti A, De Rossi D, et al. Microsyringebased deposition of two-dimensional and three-dimensional polymer scaffolds with a well-defined geometry for application to tissue engineering. Tissue Eng. 2002;8(6):10891098. https://dx.doi.org/10.1089/107632702320934182. [PubMed] [Google Scholar]
47. Tartarisco G, Gallone G, Carpi F, et al. Polyurethane unimorph bender microfabricated with pressure assisted microsyringe (PAM) for biomedical applications. Mater Sci Eng C Mater Biol Appl. 2009;29(6):1835–1841. https://dx.doi. org/10.1016/j.msec.2009.02.017. [Google Scholar]
48. Xiong Z, Yan Y, Wang S, et al. Fabrication of porous scaffolds for bone tissue engineering via low-temperature deposition. Scr Mater. 2002;46(11):771–776. https://dx.doi. org/10.1016/S1359-6462(02)00071-4. [Google Scholar]
49. Liu L, Xiong Z, Zhang R, et al. A novel osteochondral scaffold fabricated via multi-nozzle low-temperature deposition manufacturing. J Bioact Compat Polym. 2009;24(1):18–30. https://dx.doi.org/10.1177/0883911509102347. [Google Scholar]
50. Vadnere M, Amidon G, Lindenbaum S, et al. Thermodynamic studies on the gel-sol transition of some pluronic polyols. Int J Pharm. 1984;22(2-3):207–218. https:// dx.doi.org/10.1016/0378-5173(84)90022-X. [Google Scholar]
51. Kim J Y, Cho D-W. Blended PCL/PLGA scaffold fabrication using multi-head deposition system. Microelectron Eng. 2009;86(4):1447–1450. https://dx.doi. org/10.1016/ j.mee.2008.11.026. [Google Scholar]
52. Domingos M, Dinucci D, Cometa S, et al. Polycaprolactone scaffolds fabricated via bioextrusion for tissue engineering applications. Int J Biomater. 2009;2009(1687-8787):239643. https:// dx.doi.org/10.1155/2009/239643. [PMC free article] [PubMed] [Google Scholar]
53. Lam C, Olkowski R, Swieszkowski W, et al. Mechanical and in vitro evaluations of composite PLDLLA/TCP scaffolds for bone engineering. Virtual Phys Prototyp. 2008;3(4):193–197. ttps://dx.doi.org/10.1080/17452750802551298. [Google Scholar]
54. Lim T, Bang C, Chian K, et al. Development of cryogenic prototyping for tissue engineering. Virtual Phys Prototyp. 2008;3(1):25–31. https://dx.doi.org/10.1080/17452750701799303. [Google Scholar]
55. Bang Pham C, Fai Leong K, Chiun Lim T, et al. Rapid freeze prototyping technique in bio-plotters for tissue scaffold fabrication. Rapid Prototyp J. 2008;14(4):246–253. https://dx.doi.org/10.1108/13552540810896193. [Google Scholar]
56. Lu L, Zhang Q, Wootton D, et al. A novel sucrose porogen-based solid freeform fabrication system for bone scaffold manufacturing. Rapid Prototyp J. 2010;16(5):365–376. https://dx.doi.org/10.1108/13552541011065768. [Google Scholar]
57. Cima M, Sachs E, Fan T, et al. Three-dimensional printing techniques. US patent 53∢0, 1995 July 2 [Google Scholar]
58. Mei J, Lovell M, Rand Mickle M H. Formulation and processing of novel conductive solution inks in continuous inkjet printing of 3-D electric circuits. IEEE Trans Compon Packaging Manuf Technol. 2005;28(3):265–273. https://dx.doi. org/10.1109/TEPM.2005.852542. [Google Scholar]
59. Saunders R E, Gough J E, Derby B. Delivery of human fibroblast cells by piezoelectric drop-on-demand inkjet printing. Biomaterials. 2008;29(2):193–203. https://dx.doi. org/10.1016/j.biomaterials.2007.09.032. [PubMed] [Google Scholar]
60. Nakamura M, Kobayashi A, Takagi F, et al. Biocompatible inkjet printing technique for designed seeding of individual living cells. Tissue Eng. 2005;11(11-12):1658–1666. https://dx.doi. org/10.1089/ten.2005.11.1658. [PubMed] [Google Scholar]
61. Cui X, Dean D, Ruggeri Z M, et al. Cell damage evaluation of thermal inkjet printed Chinese hamster ovary cells. Biotechnol Bioeng. 2010;106(6):963–969. https://dx.doi. org/10.1002/bit.22762. [PubMed] [Google Scholar]
62. Leukers B, Gülkan H, Irsen S H, et al. Hydroxyapatite scaffolds for bone tissue engineering made by 3D printing. J MATER SCI-MATER M. 2005;16(12):1121–1124. https://dx.doi. org/10.1007/s10856-005-4716-5. [PubMed] [Google Scholar]
63. Inzana J A, Olvera D, Fuller S M, et al. 3D printing of composite calcium phosphate and collagen scaffolds for bone regeneration. Biomaterials. 2014;35(13):4026–4034. https:// dx.doi.org/10.1016/j.biomaterials.2014.01.064. [PMC free article] [PubMed] [Google Scholar]
64. Levy A, Miriyev A, Elliott A, et al. Additive manufacturing of complex-shaped graded TiC/steel composites. Mater Design. 2017;118:198–203. https://dx.doi.org/10.1016/j.matdes.2017.01.024. [Google Scholar]
65. Pfister A, Landers R, Laib A, et al. Biofunctional rapid prototyping for tissue-engineering applications:3D bioplotting versus 3D printing. J Polym Sci Pol Chem. 2004;42(3):624–638. https:// dx.doi.org/10.1002/pola.10807. [Google Scholar]
66. Boland T, Tao X, Damon B J, et al. Drop-on-demand printing of cells and materials for designer tissue constructs. Mater Sci Eng C Mater Biol Appl. 2007;27(3):372–376. https:// dx.doi.org/10.1016/j.msec.2006.05.047. [Google Scholar]
67. Sun J, Ng J H, Fuh Y H, et al. Comparison of micro-dispensing performance between micro-valve and piezoelectric printhead. Microsyst Technol. 2009;15(9):14371448. https:// dx.doi.org/10.1007/s00542-009-0905-3. [Google Scholar]
68. Zustiak S P, Leach J B. Hydrolytically degradable poly (ethylene glycol) hydrogel scaffolds with tunable degradation and mechanical properties. Biomacromolecules. 2010;11(5):1348–1357. https:// dx. doi.org/10.1021/bm100137q. [PMC free article] [PubMed] [Google Scholar]
69. Killion J A, Geever L M, Devine D M, et al. Compressive strength and bioactivity properties of photopolymerizable hybrid composite hydrogels for bone tissue engineering. Int J Polym Mater Po. 2014;63(13):641–650. https:// dx.doi.org/10.1080/00914037. 2013.⅞38. [Google Scholar]
70. Bakarich S E, Gorkin R, Gately R, et al. 3D printing of tough hydrogel composites with spatially varying materials properties. Addit Manuf. 2017;14:24–30. https:// dx.doi. org/10.1016/j.addma.2016.12.003. [Google Scholar]
71. Zhao L, Lee V K, Yoo S-S, et al. The integration of 3-D cell printing and mesoscopic fluorescence molecular tomography of vascular constructs within thick hydrogel scaffolds. Biomaterials. 2012;33(21):5325–5332. http://dx.doi.org/10.1016/j.biomaterials.2012.04.004. [PMC free article] [PubMed] [Google Scholar]
72. Hong S, Sycks D, Chan H F, et al. 3D printing of highly stretchable and tough hydrogels into complex, cellularized structures. Adv Mater. 2015;27(27):4035–4040. http://dx.doi.org/10.1002/adma.201501099. [PMC free article] [PubMed] [Google Scholar]
73. Markstedt K, Mantas A, Tournier I, et al. 3D bioprinting human chondrocytes with nanocellulose-alginate bioink for cartilage tissue engineering applications. Biomacromolecules. 2015;16(5):1489–1496. http://dx.doi.org/10.1021/acs.biomac.5b00188. [PubMed] [Google Scholar]
74. Rutz A L, Hyland K E, Jakus A E, et al. A multimaterial bioink method for 3D printing tunable, cell-compatible hydrogels. AdvMater. 2015;27(9):1607–1614. http://dx.doi.org/10.1002/adma.201405076. [PMC free article] [PubMed] [Google Scholar]
75. Xu M, Wang X, Yan Y, et al. An cell-assembly derived physiological 3D model of the metabolic syndrome, based on adipose-derived stromal cells and a gelatin/alginate/fibrinogen matrix. Biomaterials. 2010;31(14):3868–3877. http://dx.doi.org/10.1016/j.biomaterials.2010.01.111. [PubMed] [Google Scholar]
76. Akkineni A R, Ahlfeld T, Funk A, et al. Highly concentrated alginate-gellan gum composites for 3D plotting of complex tissue engineering scaffolds. Polymers. 2016;8(5):170. http://dx.doi.org/10.3390/polym8050170. [PMC free article] [PubMed] [Google Scholar]
77. Boere K W, Blokzijl M M, Visser J, et al. Biofabrication of reinforced 3D-scaffolds using two-component hydrogels. J Mater Chem B Mater Biol Med. 2015;3(46):9067–9078. http://dx.doi.org/10.1039/C5TB01645B. [PMC free article] [PubMed] [Google Scholar]
78. Censi R, Schuurman W, Malda J, et al. A printable photopolymerizable thermosensitive p (HPMAm-lactate)PEG hydrogel for tissue engineering. Adv Funct Mater. 2011;21(10):1833–1842. http://dx.doi.org/10.1002/adfm.201002428. [Google Scholar]
79. Osterbur L. 3D printing of hyaluronic acid scaffolds for tissue engineering applications [Internet] 2013 Available from: http://hdl.handle.net/2142/44207 .
80. Wang X, Cui T, Yan Y, et al. Peroneal nerve regeneration using a unique bilayer polyurethane-collagen guide conduit. J Bioact Compat Polym. 2009;24(2):109–127. http://dx.doi.org/10.1177/0883911508101183. [Google Scholar]
81. Mogas-Soldevila L, Duro-Royo J, Oxman N. Water-based robotic fabrication:Large-Scale additive manufacturing of functionally graded hydrogel composites via multichamber extrusion. 3D Print Addit Manuf. 2014;1(3):141–151. http://dx.doi.org/10.1089/3dp.2014.0014. [Google Scholar]
82. Shie M-Y, Chang W-C, Wei L-J, et al. 3D printing of cytocompatible water-based light-cured polyurethane with hyaluronic acid for cartilage tissue engineering applications. Materials. 2017;10(2):136. http://dx.doi.org/10.3390/ ma10020136. [PMC free article] [PubMed] [Google Scholar]
83. Wang X H, Tolba E, Schroder H C, et al. Effect of bioglass on growth and biomineralization of Saos-2 cells in hydrogel after 3D cell bioprinting. Plos One. 2014;9(11):e112497. http://dx.doi.org/10.1371/journal.pone.0112497. [PMC free article] [PubMed] [Google Scholar]
84. Sayyar S, Gambhir S, Chung J, et al. 3D printable conducting hydrogels containing chemically converted graphene. Nanoscale. 2017;9(5):2038–2050. http://dx.doi.org/10.1039/c6nr07516a. [PubMed] [Google Scholar]
85. Demirtas T T, Irmak G, Gumusderelioglu M. A bioprintable form of chitosan hydrogel for bone tissue engineering. Biofabrication. 2017;9(3):035003. http://dx.doi.org/10.1088/1758-5090/Aa7b1d. [PubMed] [Google Scholar]
86. Skardal A, Zhang J X, McCoard L, et al. Dynamically crosslinked gold nanoparticle-Hyaluronan hydrogels. Adv Mater. 2010;22(42):4736. http://dx.doi.org/10.1002/adma.201001436. [PubMed] [Google Scholar]
87. Fedorovich N E, Wijnberg H M, Dhert W J, et al. Distinct tissue formation by heterogeneous printing of osteoand endothelial progenitor cells. Tissue Eng Part A. 2011;17(15-16):2113–2121. http://dx.doi.org/10.1089/ten.tea.2011.0019. [PubMed] [Google Scholar]
88. Panhuis M I H, Heurtematte A, Small W R, et al. Inkjet printed water sensitive transparent films from natural gum-carbon nanotube composites. Soft Matter. 2007;3(7):840843. http://dx.doi.org/10.1039/b704368f. [PubMed] [Google Scholar]
89. Heo D N, Castro N J, Lee S J, et al. Enhanced bone tissue regeneration using a 3D printed microstructure incorporated with a hybrid nano hydrogel. Nanoscale. 2017;9(16):5055–5062. http://dx.doi.org/10.1039/c6nr09652b. [PMC free article] [PubMed] [Google Scholar]
90. Zhu W, Holmes B, Glazer R I, et al. 3D printed nanocomposite matrix for the study of breast cancer bone metastasis. Nanomedicine. 2016;12(1):69–79. http://dx.doi.org/10.1016/j.nano.2015.09.010. [PubMed] [Google Scholar]
91. Castro N J, O'Brien J, Zhang L G. Integrating biologically inspired nanomaterials and table-top stereolithography for 3D printed biomimetic osteochondral scaffolds. Nanoscale. 2015;7(33):14010–14022. http://dx.doi.org/10.1039/c5nr03425f. [PMC free article] [PubMed] [Google Scholar]
92. Gladman A S, Matsumoto E A, Nuzzo R G, et al. Biomimetic 4D printing. Nat Mater. 2016;15(4):413–418. http://dx. doi.org/10.1038/NMAT4544. [PubMed] [Google Scholar]
93. Narayanan L K, Huebner P, Fisher M B, et al. 3D-Bioprinting of polylactic acid (PLA) nanofiber-alginate hydrogel bioink containing human adipose-derived stem cells. ACS Biomater Sci Eng. 2016;2(10):1732–1742. http://dx.doi.org/10.1021/acsbiomaterials.6b00196. [Google Scholar]
94. Agrawal A, Rahbar N, Calvert P D. Strong fiberreinforced hydrogel. Acta Biomaterialia. 2013;9(2):5313–5318. http://dx.doi.org/10.1016/j.actbio.2012.10.011. [PubMed] [Google Scholar]
95. Bakarich S E, Gorkin R, Panhuis M I H, et al. Threedimensional printing fiber reinforced hydrogel composites. ACS Appl Mater Interfaces. 2014;6(18):15998–16006. http://dx.doi.org/10.1021/am503878d. [PubMed] [Google Scholar]
96. Jin Y, Liu C, Chai W, et al. Self-Supporting nanoclay as internal scaffold mterial for direct printing of soft hydrogelcomposite structures in air. ACS Appl Mater Interfaces. 2017;9(20):17456–17465. http://dx.doi.org/10.1021/acsami. 7b03613. [PubMed] [Google Scholar]
97. Zhai X, Ma Y, Hou C, et al. 3D-printed high strength bioactive supramolecular polymer/clay nanocomposite hydrogel scaffold for bone regeneration. ACS Biomater Sci Eng. 2017;3(6):1109–1118. http://dx.doi.org/10.1021/acsbiomaterials.7b00224. [Google Scholar]
98. Ahlfeld T, Cidonio G, Kilian D, et al. Development of a clay based bioink for 3D cell printing for skeletal application. Biofabrication. 2017;9(3) http://dx.doi.org/10.1088/1758-5090/aa7e96. [PubMed] [Google Scholar]
99. Egorov A A, Fedotov A Y, Mironov A V, et al. 3D printing of mineral-polymer bone substitutes based on sodium alginate and calcium phosphate. Beilstein J Nanotechnol. 2016;7(1):1794–1799. http://dx.doi.org/10.3762/bjnano.7.172. [PMC free article] [PubMed] [Google Scholar]
100. Rawat K, Agarwal S, Tyagi A, et al. Aspect ratio dependent cytotoxicity and antimicrobial properties of nanoclay. ApplBiochem Biotechnol. 2014;174(3):936–944. http://dx.doi.org/10. 1007/s12010-014-0983-2. [PubMed] [Google Scholar]
101. Mourchid A, Delville A, Lambard J, et al. Phase diagram of colloidal dispersions of anisotropic charged particles:Equilibrium properties, structure, and rheology of laponite suspensions. Langmuir. 1995;11(6):1942–1950. http://dx.doi.org/10.1021/la00006a020. [Google Scholar]
102. Su D, Jiang L, Chen X, et al. Enhancing the gelation and bioactivity of injectable silk fibroin hydrogel with laponite nanoplatelets. ACS Appl Mater Interfaces. 2016;8(15):9619–9628. http://dx.doi.org/10.1021/acsami.6b00891. [PubMed] [Google Scholar]
103. Liu Y, Meng H, Konst S, et al. Injectable dopaminemodified poly (ethylene glycol) nanocomposite hydrogel with enhanced adhesive property and bioactivity. ACS Appl Mater Interfaces. 2014;6(19):16982–16992. http://dx.doi.org/10.1021/am504566v. [PMC free article] [PubMed] [Google Scholar]
104. Demirtaş T T, Irmak G, Gümüşderelioğlu M. A bioprintable form of chitosan hydrogel for bone tissue engineering. Biofabrication. 2017;9(3):035003. http://dx.doi.org/10.1088/1758-5090/aa7b1d. [PubMed] [Google Scholar]
105. Diogo G, Gaspar V, Serra I, et al. Manufacture of β-TCP/alginate scaffolds through a Fab@home model for application in bone tissue engineering. Biofabrication. 2014;6(2):025001. http://dx.doi.org/10.1088/1758-5082/6/2/025001. [PubMed] [Google Scholar]
106. Kang M-H, Jang T-S, Jung H-D, et al. Poly (ether imide)-silica hybrid coatings for tunable corrosion behavior and improved biocompatibility of magnesium implants. Bioact Mater. 2016;11(3):035003. http://dx.doi.org/10.1088/1748-6041/11/3/035003. [PubMed] [Google Scholar]
107. Lee H, Kim Y, Kim S, et al. Mineralized biomimetic collagen/alginate/silica composite scaffolds fabricated by a low-temperature bio-plotting process for hard tissue regeneration:fabrication, characterisation and in vitro cellular activities. J Mater Chem B Mater Biol Med. 2014;2(35):5785–5798. http://dx.doi.org/10.1039/C4TB00931B. [PubMed] [Google Scholar]
108. Wang X, Tolba E, Schröder H C, et al. Effect of bioglass on growth and biomineralization of SaOS-2 cells in hydrogel after 3D cell bioprinting. PLoS One. 2014;9(11):e112497. http://dx.doi.org/10.1371/journal.pone.0112497. [PMC free article] [PubMed] [Google Scholar]
109. Huey D J, Hu J C, Athanasiou K A. Unlike bone, cartilage regeneration remains elusive. Science. 2012;338(6109):917–921. http://dx.doi.org/10.1126/science.1222454. [PMC free article] [PubMed] [Google Scholar]
110. Bartnikowski M, Akkineni A R, Gelinsky M, et al. A hydrogel model incorporating 3D-plotted hydroxyapatite for osteochondral tissue engineering. Materials. 2016;9(4):285. http://dx.doi.org/10.3390/ma9040285. [PMC free article] [PubMed] [Google Scholar]
111. Kundu J, Shim J H, Jang J, et al. An additive manufacturingbased PCL-alginate-chondrocyte bioprinted scaffold for cartilage tissue engineering. J Tissue Eng Regen Med. 2015;9(11):1286–1297. http://dx.doi. org/10.1002/term.1682. [PubMed] [Google Scholar]
112. Xu T, Binder K W, Albanna M Z, et al. Hybrid printing of mechanically and biologically improved constructs for cartilage tissue engineering applications. Biofabrication. 2012;5(1):015001. http://dx.doi.org/10.1088/1758-5082/5/1/015001. [PubMed] [Google Scholar]
113. Wei J, Wang J, Su S, et al. 3D printing of an extremely tough hydrogel. RSC Adv. 2015;5(99):81324–81329. http://dx.doi.org/10.1039/C5RA16362E. [Google Scholar]
114. Sugihara H, Toda S, Miyabara S, et al. Reconstruction of the skin in three-dimensional collagen gel matrix culture. In Vitro Cell Dev Biol Anim. 1991;27(2):142–146. http://dx.doi.org/10.1007/BF02631000. [PubMed] [Google Scholar]
115. Dorsett-Martin W A. Rat models of skin wound healing:A review. Wound Repair Regen. 2004;12(6):591–599. http://dx.doi.org/10.1111/j.1067-1927.2004.12601.x. [PubMed] [Google Scholar]
116. Skardal A, Mack D, Kapetanovic E, et al. Bioprinted amniotic fluid-derived stem cells accelerate healing of large skin wounds. Stem Cells TranslMed. 2012;1(11):792–802. http://dx.doi.org/10.5966/sctm.2012-0088. [PMC free article] [PubMed] [Google Scholar]
117. Sayyar S, Murray E, Thompson B, et al. Processable conducting graphene/chitosan hydrogels for tissue engineering. J Mater Chem B Mater Biol Med. 2015;3(3):481490. http://dx.doi.org/10.1039/C4TB01636J. [PubMed] [Google Scholar]
118. Suh J-K, Matthew H W. Application of chitosanbased polysaccharide biomaterials in cartilage tissue engineering:A review. Biomaterials. 2000;21(24):2589–2598. http://dx.doi.org/10.1016/S0142-9612(00)00126-5. [PubMed] [Google Scholar]
119. Knowlton S, Yenilmez B, Anand S, et al. Photocrosslinkingbased bioprinting:Examining crosslinking schemes. Bioprinting. 2017;5:10–18. https:// dx.doi.org/10.1016/j.bprint.2017.03.001. [Google Scholar]
120. Nair K, Gandhi M, Khalil S, et al. Characterization of cell viability during bioprinting processes. Biotechnol J. 2009;4(8):1168–1177. http://dx.doi.org/10.1002/biot. 200900004. [PubMed] [Google Scholar]
121. Arslan-Yildiz A, El Assal R, Chen P, et al. Towards artificial tissue models:Past, present, and future of 3D bioprinting. Biofabrication. 2016;8(1):014103. http://dx.doi.org/10.1088/1758-5090/8/1/014103. [PubMed] [Google Scholar]
122. Pereira R F, Bartolo P J. 3D bioprinting of photocrosslinkable hydrogel constructs. J Appl Polym Sci. 2015;132(48):42458. http://dx.doi.org/10.1002/App.42458. [Google Scholar]
123. Kirchmajer D M, Gorkin R, Panhuis M I H. An overview of the suitability of hydrogel-forming polymers for extrusion-based 3D-printing. J Mater Chem B Mater Biol Med. 2015;3(20):4105–4117. http://dx.doi.org/10.1039/c5tb00393h. [PubMed] [Google Scholar]
124. Chirag Khatiwala R L, Benjamin Shepherd, Scott Dorfman, et al. 3D cell bioprinting for regenerative medicine research and therapies. Gene Ther Regul. 2012;7(1):1230004. http://dx.doi.org/10.1142/S156855↣000301. [Google Scholar]
125. Wang Z J, Jin X, Dai R, et al. An ultrafast hydrogel photocrosslinking method for direct laser bioprinting. RSC Adv. 2016;6(25):21099–21104. http://dx.doi.org/10.1039/c5ra24910d. [Google Scholar]
126. Armstrong J P K, Burke M, Carter B M, et al. 3D bioprinting using a templated porous bioink. Adv Healthc Mater. 2016;5(14):1724–1730. http://dx.doi.org/10.1002/adhm.201600022. [PubMed] [Google Scholar]
127. Cui X F, Breitenkamp K, Finn M G, et al. Direct human cartilage repair using three-dimensional bioprinting technology. Tissue Eng Part A. 2012;18(11 -12):1304–1312. http://dx.doi.org/10.1089/ten.tea.2011.0543. [PMC free article] [PubMed] [Google Scholar]
128. Fedorovich N E, Oudshoorn M H, van Geemen D, et al. The effect of photopolymerization on stem cells embedded in hydrogels. Biomaterials. 2009;30(3):344–353. http://dx.doi.org/10.1016/j.biomaterials.2008.09.037. [PubMed] [Google Scholar]
129. Folkman J, Hochberg M. Self-regulation of growth in three dimensions. J Exp Med. 1973;138(4):745–753. [PMC free article] [PubMed] [Google Scholar]
130. Li S, Xiong Z, Wang X, et al. Direct fabrication of a hybrid cell/hydrogel construct by a double-nozzle assembling technology. J Bioact Compat Polym. 2009;24(3):249265. http://dx.doi.org/10.1016/j.biomaterials.2016.07.038. [Google Scholar]
131. Jia W, Gungor-Ozkerim P S, Zhang Y S, et al. Direct 3D bioprinting of perfusable vascular constructs using a blend bioink. Biomaterials. 2016;106:58–68. http://dx.doi.org/10.1016/j.biomaterials.2016.07.038. [PMC free article] [PubMed] [Google Scholar]
132. Skardal A, Zhang J, Prestwich G D. Bioprinting vessellike constructs using hyaluronan hydrogels crosslinked with tetrahedral polyethylene glycol tetracrylates. Biomaterials. 2010;31(24):6173–6181. http://dx.doi.org/10.1016/j.biomaterials.2010.04.045. [PubMed] [Google Scholar]
133. Dolati F, Yu Y, Zhang Y, et al. In vitro evaluation of carbon-nanotube-reinforced bioprintable vascular conduits. Nanotechnology. 2014;25(14):145101. http://dx.doi.org/10.1088/0957-4484/25/14/145101. [PMC free article] [PubMed] [Google Scholar]
134. Gao B, Yang Q Z, Zhao X, et al. 4D bioprinting for biomedical applications. Trends Biotechnol. 2016;34(9):746–756. http://dx.doi.org/10.10164.tibtech.2016.03.004. [PubMed] [Google Scholar]
135. Weiss R A, Izzo E, Mandelbaum S. New design of shape memory polymers:Mixtures of an elastomeric ionomer and low molar mass fatty acids and their salts. Macromolecules. 2008;41(9):2978–2980. http://dx.doi.org/10.1021/ma8001774. [Google Scholar]
136. Leist S K, Zhou J. Current status of 4D printing technology and the potential of light-reactive smart materials as 4D printable materials. Virtual Phys Prototyp. 2016;11(4):249262. http://dx.doi.org/10.1080/17452759.2016.119↶. [Google Scholar]
137. He H Y, Guan J J, Lee J L. An oral delivery device based on self-folding hydrogels. J Control Release. 2006;110(2):339–346. http://dx.doi.org/10.1016/jjconrel.2005.10.017. [PubMed] [Google Scholar]
138. Khoo Z X, Teoh J E M, Liu Y, et al. 3D printing of smart materials:A review on recent progresses in 4D printing. Virtual Phys Prototyp. 2015;10(3):103–122. http://dx.doi.org/10.1080/17452759.2015.1097054. [Google Scholar]
139. He Y, Wu Y, Fu J Z, et al. Developments of 3D printing microfluidics and applications in chemistry and biology:A review. Electroanalysis. 2016;28(8):1658–1678. http://dx.doi.org/10.1002/elan.201600043. [Google Scholar]
140. Lee V K, Lanzi A M, Ngo H, et al. Generation of multi-scale vascular network system within 3D hydrogel using 3D bio-printing technology. Cell Mol Bioeng. 2014;7(3):460–472. http://dx.doi.org/10.1007/s12195-014-0340-0. [PMC free article] [PubMed] [Google Scholar]
3D printing of high-strength chitosan hydrogel scaffolds without any organic solvents
Luyu Zhou, ‡ab Hamed Ramezani,‡ab Miao Sun,c Mingjun Xie,ab Jing Nie,ab Shang Lv,ab Jie Cai, d Jianzhong Fu*ab and Yong He *ab
Author affiliations
* Corresponding authors
a
State Key Laboratory of Fluid Power and Mechatronic Systems, School of Mechanical Engineering, Zhejiang University, Hangzhou 310027, China
E-mail:
fjz@zju. edu.cn, [email protected]
b Key Laboratory of 3D Printing Process and Equipment of Zhejiang Province, School of Mechanical Engineering, Zhejiang University, Hangzhou 310027, China
c Department of Oral and Maxillofacial Surgery, Affiliated Stomatology Hospital, School of Medicine, Zhejiang University, Hangzhou 310000, China
d College of Chemistry & Molecular Sciences, Wuhan University, Wuhan 430072, China
Abstract
3D printing of chitosan hydrogels has attracted wide interest because of their excellent biocompatibility, antibacterial activities, biodegradability, zero toxicity and low cost. However, chitosan inks are often involved in toxic and organic solvents. Moreover, the recently reported 3D-printed chitosan scaffolds lack enough strength, thus limiting their use in tissue engineering. Here, we reported a chitosan ink obtained by dissolving chitosan into an alkali aqueous solution. This chitosan ink is a stable solution at low temperature (5 °C), but once heated, the chitosan chains self-assemble to lead to gelation. Based on this principle, a corresponding direct ink writing (DIW) method was developed to print high-strength chitosan hydrogels. Specifically, the chitosan ink was extruded into heated deionized water to complete the in situ gelation. The temperature of the nozzle and hot water was well controlled to keep the printing process stable. The rheological behavior of the chitosan ink was investigated and the printing parameters were systematically studied to print chitosan hydrogel scaffolds with high quality and high strength. Based on these, high-strength (2.31 MPa for compressive strength) and complex chitosan hydrogel structures can be directly printed. The cell culture and the wound healing results further show that the printed chitosan scaffolds with this method have great potential in tissue engineering.
hybrid hydrogel and extrusion 3D printing / Sudo Null IT News For a long time, 3D printers were used exclusively for the production of functional or aesthetic prototypes, and the technology itself was called "rapid prototyping". The rapid development of computing technology has led to the emergence of various methods for implementing additive technologies: from laser stereolithography (SLA) to the more famous 3D printing (3DP). Another term that appeared as early as 1894, this is a hydrogel - a polymer capable of absorbing water (if very exaggerated). Hydrogels, like additive technologies, have many applications: medicine, pharmacology, and even energy.
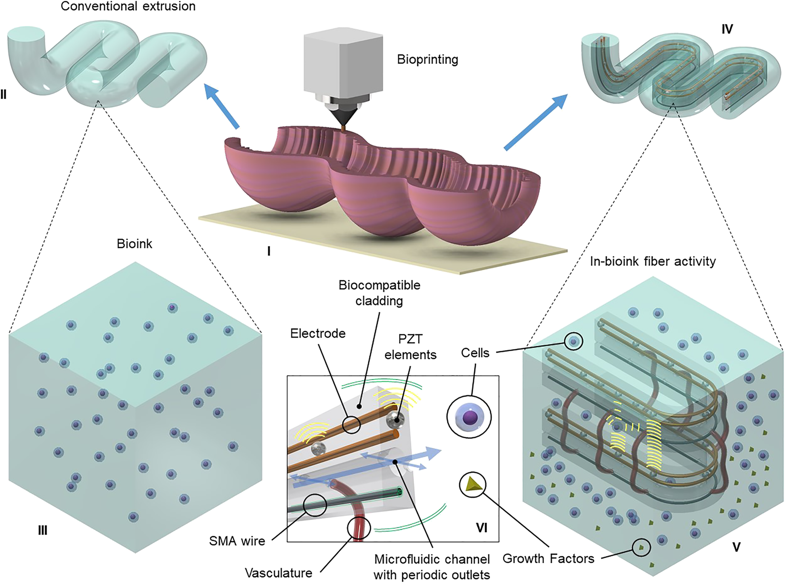
Research basis
To begin with, it is worth briefly explaining what a hydrogel is. This is a network of intersecting polymer chains capable of equilibrium and reversible swelling in water and aqueous solutions. The basis of the hydrogel are hydrophilic molecules.
The problem with classical hydrogels made from polymer networks in water is that they are soft and brittle; they lack elasticity and strength. Because of this, the use of hydrogels in various industries (robotics, tissue engineering, etc.) is severely limited. nine0003
The elasticity of hydrogel materials can be improved by combining interpenetrating covalent and ionic polymer networks to form highly extensible and strong structures. Another method for improving the mechanical properties of hydrogels is based on the use of fillers with a high aspect ratio (high ratio of the length of the filler to the diameter of its cross section). This allows the gel matrix to be mechanically strengthened. However, the use of a filler material other than the matrix material results in stresses on the interface surfaces that cause cracking when deformed or heated. nine0003
The opposite method is based on the use of single-polymer composites or so-called homocomposites. The mesoscale amplifying network of homocomposites is made from a material that is chemically identical to the primary matrix material. Networks of homocomposite reinforcement make it possible to modulate the mechanical properties of the primary (main) matrix without stress, delamination points, etc. It sounds very promising, but there is also a problem - the manufacture of HHG (from homocomposite hydrogel , i.e. homocomposite hydrogel) is an extremely difficult process due to the lack of methods for creating reinforcing networks with the same chemical composition as the hydrogel matrix. nine0003
In the work we are considering today, scientists describe a new type of HHG, in which both the primary gel matrix and the reinforcing network consist of sodium alginate (SA from sodium alginate ; C 6 H 9 NaO 7 ). These HHGs are reinforced with a fibrillar network of alginate soft dendritic colloids (SDC from soft dendritic colloid ). SDC is a hierarchically structured class of soft matter synthesized through a shear-induced deposition process in a turbulent medium. nine0003
The high degree of branching around the cores of SDC particles makes them morphologically similar to polymeric molecular dendrimers. However, SDCs are much larger than these dendrimers. Scientists believe that hierarchically branched SDCs could be an excellent option for effective reinforcement of composite materials. Significantly, the SDC branches cover a larger surface area, which can increase the stability of the composite by distributing the load more evenly.
Study results
The first step was to prepare soft alginate dendritic colloids (i.e. SDC) to serve as reinforcing meshes. For this, turbulent settling was used. To obtain an SDC hydrogel, an alginate solution (120–190 kDa) was introduced into an aqueous solution of Ca 2+ ions, which efficiently bind two -COO- side groups on the alginate backbone ( 1а ).
Image #1
The precipitation process leads to the formation of SDC with a characteristic hierarchical morphology ( 1b ) with branching of different scale and generations (secondary branches) of fibers. In fact, SDCs are made up of micron-sized fibers that fork repeatedly into ever finer fibers. The outermost layer ("crown") surrounding each SDC is made up of flexible nanofibers that can be up to 10 nm thick ( 1b ). The nanofibers in the coronas endow them with physical adhesion, which is a major factor in the ability of dendricolloids to create the structural strength of the colloidal network. The final size of conventional SDCs, including their corona, is in the range of 100–500 µm. nine0003
Next, the viscoelastic properties of aqueous SDC suspensions had to be evaluated. First, the scientists tested whether alginate SDC hydrogels form a colloidal network at low volume fractions in water. In theory, in aqueous suspensions, contacting SDCs will adhere strongly due to van der Waals forces to form a percolation* network of branched fiber subcontacts ( 2a ).
Percolation* - in chemistry, the phenomenon of the flow or non-flow of liquids through porous materials. nine0056Image #2
Evaluation of the storage moduli (G') and loss (G″) of SDC suspensions in the linear viscoelastic region showed that SDCs have a strong tendency to form colloidal networks.
Dynamic modulus* : the dynamic modulus G set can be used to represent the relationship between vibrational voltage and load: G' is the accumulation module; G″ is the loss modulus. nine0003Yield strength was observed in aqueous suspensions of 0.25 wt% SDC, i.e. at a lower concentration than most types of conventional colloidal gels.
A suspension of 1.0 wt.% SDC showed a G' value of 200 Pa, while the reported value for 1.0 wt.% alginate microgels should be 10–100 Pa. Those. SDC slurries have more pronounced solid-like characteristics than conventional alginate particle slurries.
Continuous phase HHG consists of molecular alginate gel combined with Ca 9 ions0033 2+ . First of all, the scientists analyzed the properties of molecular hydrogels containing 1.0 wt.% bound alginate, but without SDC. The hydrogel was prepared by adding CaCO 3 nanoparticles and D-gluconic acid δ-lactone (GDL) to the SA solution.
As GDL undergoes hydrolysis and lowers pH, CaCO 3 slowly releases Ca 2+ ions. After 2 hours of equilibration, data were obtained regarding the viscoelastic properties of the hydrogel ( 2c ). At a concentration of CaCO 3 above 0.05 wt.% the hydrogel behaved like a solid. With further introduction of Ca 2+ into the hydrogel, its rigidity continued to increase. But at CaCO 3 above 0.2 wt.%, syneresis (structure aging) of the hydrogel was observed, followed by water release. As a result, it was found that to maintain the stability of the hydrogel, its composition should contain 1.0 wt.% SA and 0.1 wt.% CaCO 3 .
As a result, the researchers had two components on hand that required combining - SA SDC (alginate soft dendritic colloids) and SA CMH molecular matrix (alginate gel bound by Ca 9 ions0033 2+ ).
A variety of hybrid HHGs have been synthesized where the total SA concentration is kept constant at 1 wt% and the ratio of SDC to CMH is varied.
![]()
Image #3All samples obtained in this way exhibited solids characteristics ( 3a ). The characteristic stress-strain curves ( 3b ) obtained from the mechanical tensile test also demonstrate that hybrid HHGs are more stiff than SDC or CMH gels alone. On chart 3c shows tensile-strain and rheometry measurements for all specimens. Analysis of these data shows that homocomposite systems containing mixed SDC and CMH exhibit a strong synergistic effect. The complex modulus (G) and Young's modulus (E) values for the homocomposite gels showed a threefold increase with maxima at low SDC to CMH ratios.
However, this cannot be attributed solely to the increase in the concentration of Ca 2+ in the homocomposite system. So the maximum shear modulus in HHG (G = 950 Pa at 0.125 wt. % SDC / 0.875 wt.
Therefore, a strong synergistic effect leading to an increase in the mechanical strength of HHG can be directly related to the physical interweaving of molecular SA and colloidal networks of SDC ( 3d ).
The resulting structure is stable in most environments, but can be easily destroyed by placing it in solutions of strong chelating agents such as EDTA (ethylenediaminetetraacetic acid; C 10 H 16 N 2 O 8 ).
Scientists note that another important feature of the developed hybrid hydrogel is the ability to change the kinetics of its gelation depending on.
First, the dependence of gelation on time was tested for three variants of samples: SDC, CMH and composite HHG (the SA concentration was the same for all - 1 wt.%).
Picture #4On the graph 4a presents the results of the analysis of a pure suspension of SDC. It can be seen that SDC immediately exhibits a solid behavior without the addition of CaCO 3 or GDL. This is explained by the fact that the formation of this network occurs due to contact splitting and interweaving of fibrillar dendricolloids.
On the other hand, pure CMH exhibits liquid behavior at first and gradually solidifies as the bonding agent Ca 2+ is released by hydrolysis.
The CMH becomes a fully connected structure after 120 minutes ( 4b ).
It is important to note that the time dependent evolution of HHG is directly dependent on the kinetics with which SDC and CMH (the main building blocks of HHG) are assembled in the network. HHG initially solidifies due to gelation of the mechanically rigid SDC network. A stronger hydrogel then forms as the interpenetrating CMH molecular network becomes bound by Ca 2+ ions ( 4c and 4d ).
These material properties show controlled initial yield stresses and a slow increase in hydrogel elasticity over time. Therefore, such a material can be used in 3D printing, which the scientists decided to test at the next stage of the study. nine0003
The fact that the created hydrogel is a homocomposite system allows precise control of its properties. Due to this, such a hydrogel can be used in 3D printing using extrusion, which was previously an extremely difficult task. For example, both SDC and CMH are not extrudable in their pure form, unlike hybrid HHG.
![]()
The ability to control the properties of the hydrogel allows the creation of an extrusion "ink" in which the time-independent yield strength and setting time can be adjusted to suit the individual. nine0003
Synergistic effect in mixed SA-SDC composites.
Because the 3D printer applies a pressure drop that exceeds the HHG yield strength, the extruded shape is maintained by the rapid gelation of the SDC network ( 4c , video above).
Image No. 5It is also important that the developed hydrogel could be used for printing under normal conditions without additional processing or preparation of the material ( 5а ). It is noteworthy that the G' of a pure SDC suspension (1500 Pa) is almost four orders of magnitude greater than that of the CMH mixture before the addition of GDL (0.5 Pa; 5b ).
Despite maximum HHG gel stiffness occurring at lower SDC/CMH ratios ( 4c ), HHG with higher SDC ratios produced more filaments with improved layering (video below).
![]()
3D printing of a multilayer structure by extrusion.
for 5a and 5c show the process of 3D printing a homocomposite hydrogel by direct extrusion. HHG is extruded through a nozzle (25 G, inner diameter 0.26 mm) at 140 kPa and the gel retains its shape due to its yield strength (≈80 Pa). Additional structuring in the z-direction can be achieved by stacking successive layers that have been found to adhere well to the underlying ones. The scientists were able to additively print more than 10 layers of hydrogel in the vertical direction without reducing the extrusion speed. After curing (60 minutes), the finished printed structure could be easily removed from the substrate ( 5d ). If there is a need for a structure of large dimensions, then staged extrusion is necessary, giving additional time for the gel to solidify, it is also necessary to increase the yield strength of the material by changing the composition of HHG.
![]()
For a more detailed acquaintance with the nuances of the study, I recommend looking at the report of scientists and additional materials to it.
Epilogue
In this work, scientists have demonstrated their amazing creation - a composite hydrogel, the properties of which can be manipulated depending on the needs of the end user. The hydrogel they created is great for 3D printing via extrusion, something that previous hydrogels could not boast of. nine0003
Scientists say that water-based materials are not very strong, they are brittle and soft, which is quite expected. However, if alginate soft dendritic colloids and alginate gel bound by Ca 2+ ions are combined, a hydrogel with increased strength can be obtained. In other words, they have combined two different hydrogels into one with properties that are superior to those of its constituents.
Applications for the new hydrogel include medicine, the food industry, and soft robotics.
But full use is still far away, since the hydrogel needs to be improved. In particular, the scientists want to modify the hydrogel so that it can be used in 3D printing of biomedical injection materials. nine0003
Thank you for your attention, stay curious and have a great week everyone. :)
A bit of advertising
Thank you for staying with us. Do you like our articles? Want to see more interesting content? Support us by placing an order or recommending to your friends, cloud VPS for developers from $4.99, a unique analog of entry-level servers, which was invented by us for you: The whole truth about VPS (KVM) E5-2697 v3 (6 Cores) 10GB DDR4 480GB SSD 1Gbps from $19or how to properly divide the server? (available with RAID1 and RAID10, up to 24 cores and up to 40GB DDR4).
Dell R730xd 2 times cheaper in the Maincubes Tier IV data center in Amsterdam? Only here 2 x Intel TetraDeca-Core Xeon 2x E5-2697v3 2.6GHz 14C 64GB DDR4 4x960GB SSD 1Gbps 100 TV from $199 in the Netherlands! Dell R420 - 2x E5-2430 2.
2Ghz 6C 128GB DDR3 2x960GB SSD 1Gbps 100TB - from $99! Read about How to Build Infrastructure Corp. class using Dell R730xd E5-2650 v4 servers costing 9000 euros for pennies?
3D printed hydrogel cartilage, Chinese dynamic filling algorithms and 3D printed Australian satellites
News
In this issue, we will talk about experiences in the manufacture of durable hydragel implants on desktop 3D printers, Chinese developments in creating algorithms for dynamic filling of 3D models, and the launch of three Australian satellites with a 3D printed design at once. nine0003
Hydrogel Cartilage
Researchers at Duke University have created a high-strength composite hydrogel material to mimic cartilage. The material consists of 3D printed hydrogels with different mechanical properties - one provides the necessary rigidity, and the second provides elasticity. The compressive and tensile strength indicators exceed the characteristics of standard hydrogels by about two times, while the resulting composite does not deform upon contact with water.
For the sake of the experiment, scientists printed a meniscus implant, that is, the cartilage lining of the knee joint. An important advantage of such endoprostheses will be the possibility of manufacturing on extremely cheap equipment: in the experiments, a budget desktop 3D printer costing only about $300, equipped with a syringe extruder, was used. nine0003
“We have greatly simplified the task of printing objects similar in mechanical properties to cartilage by offering a simple and inexpensive process. Available hydrogels do not reach the strength characteristics of human tissues and, as a rule, simply spread out at the outlet of the nozzle, because for the most part they consist of water. I hope this demonstration will help others interested in creating realistic and 3D printable hydrogels with mechanical properties that mimic human tissue even more closely,” said researcher Benjamin Wylie. The report of the research team is published at this link.
nine0003
Chinese version of dynamic infill
Scientists at Southeast University in Nanjing, China, have published a study to develop algorithms that optimize the infill of 3D printed models. Emphasis is placed on the selective distribution of internal structures to maximize time and material savings while maintaining or even improving strength characteristics. The report of Chinese scientists is published at this link.
Recall that similar algorithms are already available to owners of MakerBot 3D printers using the Print proprietary slicer. The update was released a month ago, and the developers promised users up to 30% material savings on average when using the MinFill dynamic filling mode. Details here. nine0003
Australia returns to space
For the first time in fifteen years, Australian-made satellites will surf the near space: yesterday, the successful launch of an Atlas 5 launch vehicle carrying payloads for the International Space Station took place.
This is even a kind of record, because before that only two Australian satellites had been in space, one of which was launched in 2002, and the first in the distant 1967. Now, three vehicles designed by scientists from the University of New South Wales went on board the ISS at once. nine0003
The launch of small satellites in the CubeSat format into free flight from the ISS will be carried out using a special installation of the NanoRacks company. Recall that additive technologies were used in the manufacture of the devices, and one of the tasks of the new Australian space program will be the testing of experimental 3D printed materials. More information about the creation and appointment of Australian satellites can be found at this link.
Do you have interesting news? Share your developments with us, and we will tell the whole world about them! We are waiting for your ideas at news@3Dtoday.
![]()
Learn more