Microgel 3d printing
Jammed Microgel-Based Inks for 3D Printing of Complex Structures Transformable via pH/Temperature Variations
. 2022 Oct;43(19):e2200271.
doi: 10.1002/marc.202200271. Epub 2022 Jun 23.
Dowon Moon 1 , Min-Gyu Lee 2 , Jeong-Yun Sun 2 3 , Kwang Hoon Song 4 , Junsang Doh 2 3 5
Affiliations
Affiliations
- 1 Department of Mechanical Engineering, Pohang University of Science and Technology (POSTECH), Pohang, Gyeongbuk, 37673, Republic of Korea.
- 2 Department of Materials Science and Engineering, Seoul National University, Seoul, 08826, Republic of Korea.
- 3 Research Institute of Advanced Materials (RIAM), Institute of Engineering Research, Seoul National University, Seoul, 08826, Republic of Korea.
- 4 Department of Nano-Bioengineering, Incheon National University, 119, Academy-ro, Yeonsu-gu, Incheon, 22012, Republic of Korea.
- 5 BioMAX Institute, Seoul National University, Seoul, 08826, Republic of Korea.
- PMID: 35686322
- DOI: 10.
1002/marc.202200271
Dowon Moon et al. Macromol Rapid Commun. 2022 Oct.
. 2022 Oct;43(19):e2200271.
doi: 10.1002/marc.202200271. Epub 2022 Jun 23.
Authors
Dowon Moon 1 , Min-Gyu Lee 2 , Jeong-Yun Sun 2 3 , Kwang Hoon Song 4 , Junsang Doh 2 3 5
Affiliations
- 1 Department of Mechanical Engineering, Pohang University of Science and Technology (POSTECH), Pohang, Gyeongbuk, 37673, Republic of Korea.
- 2 Department of Materials Science and Engineering, Seoul National University, Seoul, 08826, Republic of Korea.
- 3 Research Institute of Advanced Materials (RIAM), Institute of Engineering Research, Seoul National University, Seoul, 08826, Republic of Korea.
- 4 Department of Nano-Bioengineering, Incheon National University, 119, Academy-ro, Yeonsu-gu, Incheon, 22012, Republic of Korea.
- 5 BioMAX Institute, Seoul National University, Seoul, 08826, Republic of Korea.
- PMID: 35686322
- DOI: 10.
1002/marc.202200271
Abstract
Structure changes mediated by anisotropic volume changes of stimuli-responsive hydrogels are useful for many research fields, yet relatively simple structured objects are mostly used due to limitation in fabrication methods. To fabricate complex 3 dimensional (3D) structures that undergo structure changes in response to external stimuli, jammed microgel-based inks containing precursors of stimuli-responsive hydrogels are developed for extrusion-based 3D printing. Specifically, the jammed microgel-based inks are prepared by absorbing precursors of poly(acrylic acid) or poly(N-isopropylacrylamide) in poly(acrylamide) (PAAm) microgels, and jamming them. The inks exhibit shear-thinning and self-healing properties that allow extrusion of the inks through a nozzle and rapid stabilization after printing. Stimuli-mediated volume changes are observed for the extruded structures when they are post-crosslinked by UV light to form interpenetrating networks of PAAm microgels and stimuli-responsive hydrogels. Using this method, a dumbbell-shaped object that can transform to a biconvex shape, and a gripper that can grasp and lift an object in response to stimuli are 3D-printed. The jammed microgel-based 3D printing strategy is a versatile method useful for variety of applications as diverse types of monomers absorbable in the microgels can be used to fabricate complex 3D objects transformable by external stimuli.
Keywords: 3D printing; jamming; microgel; stimuli-responsive hydrogel.
© 2022 Wiley-VCH GmbH.
Similar articles
-
Jammed Microgel Inks for 3D Printing Applications.
Highley CB, Song KH, Daly AC, Burdick JA. Highley CB, et al. Adv Sci (Weinh). 2018 Oct 24;6(1):1801076. doi: 10.1002/advs.201801076. eCollection 2019 Jan 9. Adv Sci (Weinh). 2018.
PMID: 30643716 Free PMC article.
-
Fragmenting Bulk Hydrogels and Processing into Granular Hydrogels for Biomedical Applications.
Muir VG, Prendergast ME, Burdick JA. Muir VG, et al. J Vis Exp. 2022 May 17;(183). doi: 10.3791/63867. J Vis Exp. 2022. PMID: 35662235
-
3D Printing Method for Tough Multifunctional Particle-Based Double-Network Hydrogels.
Zhao D, Liu Y, Liu B, Chen Z, Nian G, Qu S, Yang W. Zhao D, et al. ACS Appl Mater Interfaces. 2021 Mar 24;13(11):13714-13723. doi: 10.1021/acsami.1c01413. Epub 2021 Mar 15. ACS Appl Mater Interfaces. 2021. PMID: 33720679
-
Composite Inks for Extrusion Printing of Biological and Biomedical Constructs.
Ravanbakhsh H, Bao G, Luo Z, Mongeau LG, Zhang YS. Ravanbakhsh H, et al. ACS Biomater Sci Eng. 2021 Sep 13;7(9):4009-4026. doi: 10.1021/acsbiomaterials.0c01158. Epub 2020 Nov 10. ACS Biomater Sci Eng. 2021. PMID: 34510905 Review.
-
Smart and Biomimetic 3D and 4D Printed Composite Hydrogels: Opportunities for Different Biomedical Applications.
Malekmohammadi S, Sedghi Aminabad N, Sabzi A, Zarebkohan A, Razavi M, Vosough M, Bodaghi M, Maleki H. Malekmohammadi S, et al. Biomedicines. 2021 Oct 26;9(11):1537. doi: 10.3390/biomedicines9111537. Biomedicines. 2021. PMID: 34829766 Free PMC article. Review.
See all similar articles
References
-
- M. Wei, Y. Gao, X. Li, M.
J. Serpe, Polym. Chem. 2017, 8, 127.
- M. Wei, Y. Gao, X. Li, M.
-
- A. Sosnik, D. Cohn, Biomaterials 2004, 25, 2851.
-
- Y. Zhang, J. Liao, T. Wang, W. Sun, Z. Tong, Adv. Funct. Mater. 2018, 28, 1707245.
-
- J. Li, X. Zhou, Z. Liu, Adv. Opt. Mater. 2020, 8, 2000886.
-
- S. Guo, K. Matsukawa, T. Miyata, T. Okubo, K. Kuroda, A. Shimojima, J. Am. Chem. Soc. 2015, 137, 15434.
MeSH terms
Substances
Grant support
- NRF-2020R1A2B5B03001747/the National Research Foundation of Korea (NRF)
- NRF-2021R1C1C1010633/the National Research Foundation of Korea (NRF)
- 2019-0363/Incheon National University (International Cooperative)
3D Printing of Microgel Scaffolds with Tunable Void Fraction to Promote Cell Infiltration
. 2021 Sep;10(18):e2100644.
doi: 10.1002/adhm.202100644. Epub 2021 Aug 3.
Alexis J Seymour 1 , Sungchul Shin 2 , Sarah C Heilshorn 2
Affiliations
Affiliations
- 1 Department of Bioengineering, Stanford University, Stanford, CA, 94305, USA.
- 2 Department of Materials Science & Engineering, Stanford University, Stanford, CA, 94305, USA.
- PMID: 34342179
- PMCID: PMC8612872
- DOI: 10.
1002/adhm.202100644
Free PMC article
Alexis J Seymour et al. Adv Healthc Mater. 2021 Sep.
Free PMC article
. 2021 Sep;10(18):e2100644.
doi: 10.1002/adhm.202100644. Epub 2021 Aug 3.
Authors
Alexis J Seymour 1 , Sungchul Shin 2 , Sarah C Heilshorn 2
Affiliations
- 1 Department of Bioengineering, Stanford University, Stanford, CA, 94305, USA.
- 2 Department of Materials Science & Engineering, Stanford University, Stanford, CA, 94305, USA.
- PMID: 34342179
- PMCID: PMC8612872
- DOI: 10.1002/adhm.202100644
Abstract
Granular, microgel-based materials have garnered interest as promising tissue engineering scaffolds due to their inherent porosity, which can promote cell infiltration. Adapting these materials for 3D bioprinting, while maintaining sufficient void space to enable cell migration, can be challenging, since the rheological properties that determine printability are strongly influenced by microgel packing and void fraction. In this work, a strategy is proposed to decouple printability and void fraction by blending UV-crosslinkable gelatin methacryloyl (GelMA) microgels with sacrificial gelatin microgels to form composite inks. It is observed that inks with an apparent viscosity greater than ≈100 Pa s (corresponding to microgel concentrations ≥5 wt%) have rheological properties that enable extrusion-based printing of multilayered structures in air. By altering the ratio of GelMA to sacrificial gelatin microgels, while holding total concentration constant at 6 wt%, a family of GelMA:gelatin microgel inks is created that allows for tuning of void fraction from 0.20 to 0.57. Furthermore, human umbilical vein endothelial cells (HUVEC) seeded onto printed constructs are observed to migrate into granular inks in a void fraction-dependent manner. Thus, the family of microgel inks holds promise for use in 3D printing and tissue engineering applications that rely upon cell infiltration.
Keywords: 3D printing; cell infiltration; endothelial cells; gelatin methacryloyl; microgels; sacrificial inks; void fractions.
© 2021 Wiley-VCH GmbH.
Figures
Figure 1.
Design of microporous GelMA microgel…
Figure 1.
Design of microporous GelMA microgel inks with sacrificial gelatin microgels. (a) Schematic illustration…
Figure 1.Design of microporous GelMA microgel inks with sacrificial gelatin microgels. (a) Schematic illustration depicting the process for producing 3D printed constructs with tunable void fraction. Gelatin and GelMA microgels are fabricated via complex coacervation method. Jammed composite inks containing a blend of crosslinkable GelMA microgels and sacrificial gelatin microgels are 3D printed, UV crosslinked, and incubated at 37°C to form constructs with tunable void space. (b) False-colored image of a representative sample of rhodamine B-stained gelatin and GelMA microgels before washing, after washing, after jamming, and after 3D printing. (c) Particle diameter of GelMA and gelatin at each stage. Data are plotted as mean ± standard deviation, n = 100 replicates. Scale bars are 100 μm in panel b. Statistical significance tested by one-way ANOVA with Tukey’s post-hoc analysis; n.s. = not significant (p > 0.05), *p < 0.05, **p < 0.01, ***p < 0.001, ****p < 0.0001.
Figure 2.
Rheological characteristics and printability of…
Figure 2.
Rheological characteristics and printability of GelMA and gelatin microgel inks without UV crosslinking.…
Figure 2.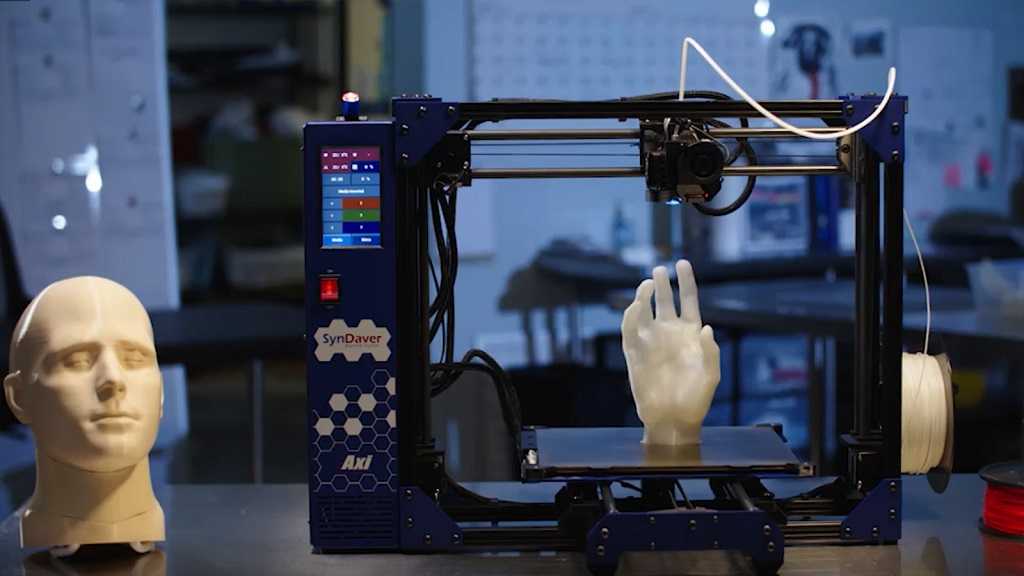
Rheological characteristics and printability of GelMA and gelatin microgel inks without UV crosslinking. (a) Storage moduli (G′, filled symbols) and loss moduli (G″, open symbols) of GelMA microgel inks with a range of total microgel concentration (2–6 wt%) as a function of shear stress (0.1–1000 Pa). (b) Shear viscosity with increasing shear rates (0.1–1000 s−1) demonstrates that GelMA microgel inks are shear-thinning. (c) Evaluation of the self-recovery of a 6-wt% GelMA microgel ink under alternating shear stress (0.1 Pa and 300 Pa). (d) Storage moduli (G′, filled symbols) and loss moduli (G″, open symbols) of composite GelMA:gelatin microgel inks with different ratios (all GelMA: 100:0; all gelatin: 0:100) as a function of shear stress (0.1–1000 Pa). (e) Shear viscosity with increasing shear rates (0.1–1000 s−1) of sacrificial gelatin microgel-laden composite inks. (f) 3D model and representative image of an uncrosslinked lattice structure 3D printed in air using a 6-wt% microgel ink. (g) Optical microscopic images of 3D printed lattice structures made using GelMA with different concentration from 2 to 6 wt% (top row) and 6 wt% GelMA:gelatin microgel composite inks (bottom row) with different ratios from 0:100 to 100:0. (h) Apparent viscosity of GelMA microgel inks at an applied shear rate of 0.1 s−1 as a function of GelMA microgel concentration, with printable (“Printed Window Remains”) and not printable (“Printed Window Disappears”) inks labeled based on printing studies shown in (g,i, and j). (i) The quantified ink spreading (Sp) and (j) window printability (Prw) of 6-wt% inks with different GelMA:gelatin microgel ratios compared to a 5-wt% microgel ink. Data in (i) and (j) plotted as a box and whisker plot, with whiskers showing the minimum and maximum values, and a superimposed scatter plot of all points; n = 36 windows. Scale bars are 10 mm in panel f and 5 mm in panel g. Statistical significance tested by one-way ANOVA with Tukey’s post-hoc analysis; n.
s. = not significant (p > 0.05), *p < 0.05, **p < 0.01, ***p < 0.001, ****p < 0.0001.
Figure 3.
Ability to print self-supporting, stacked…
Figure 3.
Ability to print self-supporting, stacked structure quantified by height maintenance, H M ,…
Figure 3.Ability to print self-supporting, stacked structure quantified by height maintenance, HM, of microgel inks. (a) A 1-cm (10 mm) tall cylinder was printed via continuous extrusion through a 27-gauge needle to investigate the ability of various microgel inks to support layer stacking, measured by height maintenance (HM). Height maintenance (HM) of uncrosslinked, 3D printed cylindrical structures made using (b) 100:0 inks with concentrations ranging from 2 to 6 wt% and (c) GelMA:gelatin microgel composite inks with ratios from 0:100 to 100:0 (n = 12 printed cylinders). (d) UV-crosslinked cylinders printed with inks of varying GelMA:gelatin ratio were filled with 37°C water to assess their ability to maintain their height after melting sacrificial gelatin microgels (n = 6 printed cylinders; additional images in Figure S6 and Figure S7, Supporting Information). Data are plotted as mean ± standard deviation. Statistical significance tested by one-way ANOVA with Tukey’s post-hoc analysis; n.s. = not significant (p > 0.05), *p < 0.05, **p < 0.01, ***p < 0.001, ****p < 0.0001.
Figure 4.
Representative images and quantification of…
Figure 4.
Representative images and quantification of void fraction in samples printed with GelMA:gelatin microgel…
Figure 4.
Representative images and quantification of void fraction in samples printed with GelMA:gelatin microgel inks after UV crosslinking and sacrificial gelatin removal. (a) Microgels are shown in black, and voids (which are filled with 2,000-kDa FITC-dextran) are shown in green. (b) Quantified void fraction for inks of varying GelMA:gelatin microgel ratios; mean ± standard deviation, n = 6. 3D printed structures demonstrating the versatility of GelMA:gelatin microgel inks. (c) A chessboard pattern was printed from two different microgel composite inks (100:0 and 40:60, visualized with red and green fluorescent microparticles, respectively). (d) After UV-crosslinking and melting of sacrificial gelatin microgels, regions of patterned void structure were observed by diffusing rhodamine B (red) into the GelMA microgels. (e) An embedded cylindrical channel was printed with two different microgel composite inks (100:0 and 0:100, visualized with red and blue fluorescent microparticles, respectively). Cross-sectional images of the embedded channel (f) pre-melt and (g) post-melt. Scale bars are 500 μm in a, 10 mm in c, 1 mm in d, and 5 mm in e-g. Statistical significance tested by one-way ANOVA with Tukey’s post-hoc analysis; *p < 0.05, **p < 0.01, ***p < 0.001, ****p < 0.0001.
Figure 5.
Shear moduli of UV-crosslinked GelMA:gelatin…
Figure 5.
Shear moduli of UV-crosslinked GelMA:gelatin inks before and after melting sacrificial gelatin microgels.…
Figure 5.Shear moduli of UV-crosslinked GelMA:gelatin inks before and after melting sacrificial gelatin microgels. (a,b) Representative shear moduli (G′, filled symbols; G″, open symbols) of UV-crosslinked GelMA:gelatin microgel inks (a) before melting (25°C) and (b) after melting (37°C) sacrificial gelatin microgels. (c) Comparison of pre- and post-melting storage moduli (G′) plotted as mean ± standard deviation, n = 3. Statistical significance was evaluated using one-way ANOVA with Tukey’s post-hoc analysis; n.s. = not significant (p > 0.05), *p < 0.05, **p < 0.01, ***p < 0.001, ****p < 0.0001.
Figure 6.
Microgel-based inks with greater void…
Figure 6.
Microgel-based inks with greater void fraction enhance cell infiltration into 3D printed constructs.…
Figure 6.Microgel-based inks with greater void fraction enhance cell infiltration into 3D printed constructs. (a) Live/Dead™ staining after 1 day of culture demonstrates that all material conditions support HUVEC viability (calcein AM = green, live; ethidium homodimer-1 = red, dead; scale bars are 250 μm). For comparison, HUVEC were also cultured on a control substrate of flat GelMA (labeled “Flat Gel”). Data are plotted as mean ± standard deviation, n = 6 images; N = 2–3 replicates. (b) Quantification of cell metabolism shows an increase in overall metabolism per gel over time in all conditions, normalized to day 1 values. Data are plotted as mean ± standard deviation, n = 6 replicates. (c) Quantification of nuclear z-position within GelMA:gelatin microgel inks demonstrating increased number of cells are found deeper within inks with greater total void fraction (n = 35–3,961 cells per condition; N = 3 sample replicates). Data are plotted as violin plots, with median and quartiles denoted by solid and dashed lines, respectively. The top surface of the construct (50 µm) is denoted by a white band. (d) Representative images of HUVEC cultured on a negative control surface of flat GelMA and GelMA:gelatin microgel inks at days 1 and 7. Actin is false-colored to denote z-position within the scaffold; nuclei are false-colored white.
Scale bars are 100 μm. Statistical significance tested by one-way ANOVA (a, c) or two-way ANOVA (b) with Tukey’s post-hoc analysis; n.s. = not significant (p > 0.05), *p < 0.05, **p < 0.01, ***p < 0.001, ****p < 0.0001.
Figure 7.
Representative projections of surface (0–50…
Figure 7.
Representative projections of surface (0–50 μm) and internal (75–150 μm) zones within 3D…
Figure 7.Representative projections of surface (0–50 μm) and internal (75–150 μm) zones within 3D printed microgel constructs after 7 days of culture. In 40:60 inks (left), cells are seen to spread along voids introduced by sacrificial gelatin microgels, allowing them to infiltrate deeper into the construct. In contrast, cells seeded onto constructs printed without sacrificial gelatin microgel (100:0, right) spread along the surface and are not found within the construct. Scale bars represent 100 μm.
See this image and copyright information in PMC
Similar articles
-
Effects of transglutaminase cross-linking process on printability of gelatin microgel-gelatin solution composite bioink.
Song K, Ren B, Zhai Y, Chai W, Huang Y. Song K, et al. Biofabrication. 2021 Dec 14;14(1). doi: 10.1088/1758-5090/ac3d75. Biofabrication. 2021. PMID: 34823234
-
Cross-Linkable Microgel Composite Matrix Bath for Embedded Bioprinting of Perfusable Tissue Constructs and Sculpting of Solid Objects.
Compaan AM, Song K, Chai W, Huang Y.
Compaan AM, et al. ACS Appl Mater Interfaces. 2020 Feb 19;12(7):7855-7868. doi: 10.1021/acsami.9b15451. Epub 2020 Feb 10. ACS Appl Mater Interfaces. 2020. PMID: 31948226
-
Embedded 3D Bioprinting of Gelatin Methacryloyl-Based Constructs with Highly Tunable Structural Fidelity.
Ning L, Mehta R, Cao C, Theus A, Tomov M, Zhu N, Weeks ER, Bauser-Heaton H, Serpooshan V. Ning L, et al. ACS Appl Mater Interfaces. 2020 Oct 7;12(40):44563-44577. doi: 10.1021/acsami.0c15078. Epub 2020 Sep 23. ACS Appl Mater Interfaces. 2020. PMID: 32966746
-
Assembling Microgels via Dynamic Cross-Linking Reaction Improves Printability, Microporosity, Tissue-Adhesion, and Self-Healing of Microgel Bioink for Extrusion Bioprinting.
Feng Q, Li D, Li Q, Li H, Wang Z, Zhu S, Lin Z, Cao X, Dong H.
Feng Q, et al. ACS Appl Mater Interfaces. 2022 Apr 6;14(13):15653-15666. doi: 10.1021/acsami.2c01295. Epub 2022 Mar 28. ACS Appl Mater Interfaces. 2022. PMID: 35344348
-
Reversible physical crosslinking strategy with optimal temperature for 3D bioprinting of human chondrocyte-laden gelatin methacryloyl bioink.
Gu Y, Zhang L, Du X, Fan Z, Wang L, Sun W, Cheng Y, Zhu Y, Chen C. Gu Y, et al. J Biomater Appl. 2018 Nov;33(5):609-618. doi: 10.1177/0885328218805864. Epub 2018 Oct 25. J Biomater Appl. 2018. PMID: 30360677
See all similar articles
Cited by
-
Frozen bean curd-inspired xenogeneic acellular dermal matrix with triple pretreatment approach of freeze-thaw, laser drilling and ADSCs pre-culture for promoting early vascularization and integration.
Huang X, Zhu Z, Lu L, Jin R, Sun D, Luo X. Huang X, et al. Regen Biomater. 2022 Aug 4;9:rbac053. doi: 10.1093/rb/rbac053. eCollection 2022. Regen Biomater. 2022. PMID: 35974951 Free PMC article.
-
4D Printing of Extrudable and Degradable Poly(Ethylene Glycol) Microgel Scaffolds for Multidimensional Cell Culture.
Miksch CE, Skillin NP, Kirkpatrick BE, Hach GK, Rao VV, White TJ, Anseth KS. Miksch CE, et al. Small. 2022 Sep;18(36):e2200951. doi: 10.1002/smll.202200951. Epub 2022 Jun 22. Small. 2022. PMID: 35732614
-
Application Status of Sacrificial Biomaterials in 3D Bioprinting.
Liu S, Wang T, Li S, Wang X. Liu S, et al. Polymers (Basel). 2022 May 27;14(11):2182. doi: 10.3390/polym14112182.
Polymers (Basel). 2022. PMID: 35683853 Free PMC article. Review.
-
Simultaneous One-Pot Interpenetrating Network Formation to Expand 3D Processing Capabilities.
Dhand AP, Davidson MD, Galarraga JH, Qazi TH, Locke RC, Mauck RL, Burdick JA. Dhand AP, et al. Adv Mater. 2022 Jul;34(28):e2202261. doi: 10.1002/adma.202202261. Epub 2022 Jun 4. Adv Mater. 2022. PMID: 35510317
-
Sticking Together: Injectable Granular Hydrogels with Increased Functionality via Dynamic Covalent Inter-Particle Crosslinking.
Muir VG, Qazi TH, Weintraub S, Torres Maldonado BO, Arratia PE, Burdick JA. Muir VG, et al. Small. 2022 Sep;18(36):e2201115. doi: 10.1002/smll.202201115. Epub 2022 Mar 22. Small. 2022. PMID: 35315233
See all "Cited by" articles
Publication types
MeSH terms
Substances
Grant support
- R01 HL142718/HL/NHLBI NIH HHS/United States
- R01 HL142718/NH/NIH HHS/United States
Engineers have mastered 3D printing with silicone inside a microgel / Sudo Null IT News
Scientists from the University of Florida have learned how to print with silicone inside a microgel. This discovery promises to significantly advance the technology of medical implants: they will become more reliable, cheaper and more convenient than all the implants available on the market today. And it's not what you think, but quite different. For example, heart valves, as well as soft catheters, drainage instruments, implantable meshes and other surgical accessories.
Currently, such devices have to be molded on presses. If a specific shape and size is required, then the procedure for waiting for the operation can be delayed for several days or even weeks. Not every patient will live to have a silicone part of the desired shape made. 3D printing reduces production time to hours, which could theoretically save someone's life.
Microgels are swollen microparticles formed from interconnected polymers. They have very specific and useful rheological properties, that is, a specific combination of deformation and fluidity. Therefore, they are manufactured on an industrial scale for use as lubricants, personal care products, to coat materials, and even to collect oil. Under the influence of extreme pressure, the microgel turns into a liquid - this does not happen spontaneously when a substance is heated. It is this property (temporary transformation into a liquid) that is used for 3D printing of liquid objects inside the microgel.
Conventional industrial production methods - swelling synthesis - have limited the scope of microgels. But a few years ago, scientists mastered 3D printing, and then a completely new hypostasis was found for this material. The squashed microgel is used to print unique hydrogel and silicone structures, and even constructs to support living cells. With this technique, the printed material is literally physically squeezed into the space of a packed microgel, which temporarily turns into a liquid under the influence of a printing (injection) tip, and then quickly returns to its previous gel-like state. Thus, it becomes possible to print soft and fragile materials inside the container with high precision. This technique was used by researchers from the University of Florida. The scientific group has been working on 3D printing of living organs and tissues for several years, and this project was a by-product of their research.
The main scientific discovery in this case is the chemical structure of the container. Previous water-based microgels did not allow printing with liquid silicone, which literally dissolved in the container. Therefore, scientists have developed an oil-based material with which the "paint" does not mix.
The production time for surgical accessories and implants is reduced by an order of magnitude, which in itself is a huge achievement. But at the same time, it also becomes possible to produce parts of a more complex shape. Some types of products, such as drainage tubes with pressure-sensitive valves, cannot be made in a single step press at all. This is complex and painstaking work. Now they can be easily printed.
Printed Silicone Objects
"Our new material provides support for liquid silicone and allows for the 3D printing of very complex structures and even encapsulated silicone elastomer parts," says lead author Christopher O'Bryan, Ph. D. doctoral student of the department of mechanical and aerospace engineering.
It's not entirely appropriate to talk about finances in this area, but accessories made in a new way should be significantly cheaper than the current ones.
Presumably, with the advent of new technology, it will even be possible to develop new therapeutic devices that can encapsulate substances - and release them in small quantities inside the body. These are drugs or molecules that stimulate tissue growth in the right places. If we dream up, then sometime in the future we will literally begin to build up muscles, blood vessels and nervous tissue on top of the skeleton - to build the human body almost from scratch, like in science fiction.
Printing organs and tissues remains the main goal of a group of researchers from the University of Florida, and they continue to experiment with 3D printers and various materials. According to engineers, this will be possible in a few decades. It's quite a distant future, so they briefly digressed to a side project of printing silicone implants, an invention that can be used today.
Scientific paper published on May 10, 2017 in Science Advances (doi:10.1126/sciadv.1602800).
An original method for high-precision 3D printing with silicone inside a microgel is proposed.
Scientists from the University of Florida have learned how to print with silicone inside a microgel. This discovery promises to significantly advance the technology of medical implants: they will become more reliable, cheaper and more convenient than all the implants available on the market today. And it's not what you think, but quite different. For example, heart valves, as well as soft catheters, drainage instruments, implantable meshes and other surgical accessories.
Take advantage of our services
These devices currently have to be molded on presses. If a specific shape and size is required, then the procedure for waiting for the operation can be delayed for several days or even weeks. Not every patient will live to have a silicone part of the desired shape made. 3D printing reduces production time to hours, which could theoretically save someone's life.
Microgels are swollen microparticles formed from interconnected polymers. They have very specific and useful rheological properties, that is, a specific combination of deformation and fluidity. Therefore, they are manufactured on an industrial scale for use as lubricants, personal care products, to coat materials, and even to collect oil. Under the influence of extreme pressure, the microgel turns into a liquid - this does not happen spontaneously when a substance is heated. It is this property (temporary transformation into a liquid) that is used for 3D printing of liquid objects inside the microgel.
Conventional industrial production methods - swelling synthesis - have limited the scope of microgels. But a few years ago, scientists mastered 3D printing, and then a completely new hypostasis was found for this material. The squashed microgel is used to print unique hydrogel and silicone structures, and even constructs to support living cells. With this technique, the printed material is literally physically squeezed into the space of a packed microgel, which temporarily turns into a liquid under the influence of a printing (injection) tip, and then quickly returns to its previous gel-like state. Thus, it becomes possible to print soft and fragile materials inside the container with high precision. This technique was used by researchers from the University of Florida. The scientific group has been working on 3D printing of living organs and tissues for several years, and this project was a by-product of their research.
The main scientific discovery in this case is the chemical structure of the container. Previous water-based microgels did not allow printing with liquid silicone, which literally dissolved in the container. Therefore, scientists have developed an oil-based material with which the "paint" does not mix.
The production time for surgical accessories and implants is reduced by an order of magnitude, which in itself is a huge achievement. But at the same time, it also becomes possible to produce parts of a more complex shape. Some types of products, such as drainage tubes with pressure-sensitive valves, cannot be made in a single step press at all. This is complex and painstaking work. Now they can be easily printed.
Printed Silicone Objects
"Our new material provides support for liquid silicone and allows for the 3D printing of very complex structures and even encapsulated silicone elastomer parts," says lead author Christopher O'Bryan, Ph.D. doctoral student of the department of mechanical and aerospace engineering.
It's not entirely appropriate to talk about finances in this area, but accessories made in a new way should be significantly cheaper than the current ones.
Presumably, with the advent of new technology, it will even be possible to develop new therapeutic devices that can encapsulate substances - and release them in small quantities inside the body. These are drugs or molecules that stimulate tissue growth in the right places. If we dream up, then sometime in the future we will literally begin to build up muscles, blood vessels and nervous tissue on top of the skeleton - to build the human body almost from scratch, like in science fiction.
Printing organs and tissues remains the primary goal of a group of researchers at the University of Florida, and they continue to experiment with 3D printers and various materials. According to engineers, this will be possible in a few decades. It's quite a distant future, so they briefly digressed to a side project of printing silicone implants, an invention that can be used today.