3D printer biological material
3D Bioprinting of Living Tissues
Progress in drug testing and regenerative medicine could greatly benefit from laboratory-engineered human tissues built of a variety of cell types with precise 3D architecture. But production of greater than millimeter sized human tissues has been limited by a lack of methods for building tissues with embedded life-sustaining vascular networks.
Play
In this video, the Wyss Institute and Harvard SEAS team uses a customizable 3D bioprinting method to build a thick vascularized tissue structure comprising human stem cells, collective matrix, and blood vessel endothelial cells. Their work sets the stage for advancement of tissue replacement and tissue engineering techniques.Multidisciplinary research at the Wyss Institute has led to the development of a multi-material 3D bioprinting method that generates vascularized tissues composed of living human cells that are nearly ten-fold thicker than previously engineered tissues and that can sustain their architecture and function for upwards of six weeks. The method uses a customizable, printed silicone mold to house and plumb the printed tissue on a chip. Inside this mold, a grid of larger vascular channels containing living endothelial cells in silicone ink is printed, into which a self-supporting ink containing living mesenchymal stem cells (MSCs) is layered in a separate print job. After printing, a liquid composed of fibroblasts and extracellular matrix is used to fill open regions within the construct, adding a connective tissue component that cross-links and further stabilizes the entire structure.
Confocal microscopy image showing a cross-section of a 3D-printed, 1-centimeter-thick vascularized tissue construct showing stem cell differentiation towards development of bone cells, following one month of active perfusion of fluids, nutrients, and cell growth factors.
The resulting soft tissue structure can be immediately perfused with nutrients as well as growth and differentiation factors via a single inlet and outlet on opposite ends of the chip that connect to the vascular channel to ensure survival and maturation of the cells. In a proof-of-principle study, one centimeter thick bioprinted tissue constructs containing human bone marrow MSCs surrounded by connective tissue and supported by an artificial endothelium-lined vasculature, allowed the circulation of bone growth factors and, subsequently, the induction of bone development.
This innovative bioprinting approach can be modified to create various vascularized 3D tissues for regenerative medicine and drug testing endeavors. The Wyss team is also investigating the use of 3D bioprinting to fabricate new versions of the Institute’s organs on chips devices, which makes their manufacturing process more automated and enables development of increasingly complex microphysiological devices. This effort has resulted in the first entirely 3D-printed organ on a chip – a heart on a chip – with integrated soft strain sensors.
- 1/7 Cross section of long-term perfusion of HUVEC-lined (red) vascular network supporting HNDFladen (green) matrix.
- 2/7 Top-down view of long-term perfusion of HUVEC-lined (red) vascular network supporting HNDFladen (green) matrix.
- 3/7 Photograph cross section of printed tissue construct housed within a perfusion chamber.
- 4/7 Photograph cross section of printed tissue construct housed within a perfusion chamber.
- 5/7 Photograph of a printed tissue construct housed within a perfusion chamber.
- 6/7 Photograph of vasculature network and cell inks.
- 7/7 Photograph of 3D printed vasculature network (red) within Red is the
- Next
- Prev
Novel Biomaterials Used in Medical 3D Printing Techniques
1. Belhabib S., Guessasma S. Compression performance of hollow structures: From topology optimisation to design 3D printing. Int. J. Mech. Sci. 2017;133:728–739. doi: 10.1016/j.ijmecsci.2017.09.033. [CrossRef] [Google Scholar]
2. Guessasma S., Nouri H., Roger F. Microstructural and Mechanical Implications of Microscaled Assembly in Droplet-based Multi-Material Additive Manufacturing. Polymers. 2017;9:372. doi: 10.3390/polym9080372. [CrossRef] [Google Scholar]
3. Ligon S.C., Liska R., Stampfl J., Gurr M., Mülhaupt R. Polymers for 3D Printing and Customized Additive Manufacturing. Chem. Rev. 2017;117:10212–10290. doi: 10.1021/acs.chemrev.7b00074. [PMC free article] [PubMed] [CrossRef] [Google Scholar]
4. Liu T., Guessasma S., Zhu J., Zhang W., Nouri H., Belhabib S. Microstructural defects induced by stereolithography and related compressive behaviour of polymers. J. Mater. Process. Technol. 2018;251:37–46. doi: 10.1016/j.jmatprotec.2017.08.014. [CrossRef] [Google Scholar]
5. Mandrycky C., Wang Z., Kim K., Kim D.H. 3D bioprinting for engineering complex tissues. Biotechnol. Adv. 2016;34:422–434. doi: 10.1016/j.biotechadv.2015.12.011. [PMC free article] [PubMed] [CrossRef] [Google Scholar]
6. Rezwan K., Chen Q.Z., Blaker J.J., Boccaccini A.R. Biodegradable and bioactive porous polymer/inorganic composite scaffolds for bone tissue engineering. Biomaterials. 2006;27:3413–3431. doi: 10.1016/j.biomaterials.2006.01.039. [PubMed] [CrossRef] [Google Scholar]
7. Godbey W.T., Atala A. In vitro systems for tissue engineering. Ann. N. Y. Acad. Sci. 2002;961:10–26. doi: 10.1111/j.1749-6632.2002.tb03041.x. [PubMed] [CrossRef] [Google Scholar]
8. Dong L., Wang S.J., Zhao X.R., Zhu Y.F., Yu J.K. 3D-printed poly (ϵ-caprolactone) scaffold integrated with cell-laden chitosan hydrogels for bone tissue engineering. Sci. Rep. 2017;7:13412. doi: 10.1038/s41598-017-13838-7. [PMC free article] [PubMed] [CrossRef] [Google Scholar]
9. Shim J.-H., Won J.-Y., Park J.-H., Bae J.-H., Ahn G., Kim C.-H., Lim D.-H., Cho D.-W., Yun W.-S., Bae E.-B., et al. Effects of 3D-Printed Polycaprolactone/β-Tricalcium Phosphate Membranes on Guided Bone Regeneration. Int. J. Mol. Sci. 2017;18:899. doi: 10.3390/ijms18050899. [PMC free article] [PubMed] [CrossRef] [Google Scholar]
10. Mills D., Tappa K., Jammalamadaka U., Weisman J., Woerner J. The Use of 3D Printing in the Fabrication of Nasal Stents. Inventions. 2017;3:1. doi: 10.3390/inventions3010001. [CrossRef] [Google Scholar]
11. Weisman J.A., Nicholson J.C., Tappa K., Jammalamadaka U., Wilson C.G., Mills D.K. Antibiotic and chemotherapeutic enhanced three-dimensional printer filaments and constructs for biomedical applications. Int. J. Nanomed. 2015;10:357–370. [PMC free article] [PubMed] [Google Scholar]
12. Tappa K., Jammalamadaka U., Ballard D.H., Bruno T., Israel M.R., Vemula H., Meacham J.M., Mills D.K., Woodard P.K., Weisman J.A. Medication eluting devices for the field of OBGYN (MEDOBGYN): 3D printed biodegradable hormone eluting constructs; a proof of concept study. PLoS ONE. 2017;12:e0182929. doi: 10.1371/journal.pone.0182929. [PMC free article] [PubMed] [CrossRef] [Google Scholar]
13. Horst D.J., Tebcherani S.M., Kubaski E.T., De Almeida Vieira R. Bioactive Potential of 3D-Printed Oleo-Gum-Resin Disks: B. papyrifera; C. myrrha; and S. benzoin Loading Nanooxides—TiO2, P25, Cu2O; and MoO3. Bioinorg. Chem. Appl. 2017;2017:6398167. doi: 10.1155/2017/6398167. [PMC free article] [PubMed] [CrossRef] [Google Scholar]
14. Weisman J., Jammalamadaka U., Tappa K., Mills D. Doped Halloysite Nanotubes for Use in the 3D Printing of Medical Devices. Bioengineering. 2017;4:96. doi: 10.3390/bioengineering4040096. [PMC free article] [PubMed] [CrossRef] [Google Scholar]
15. Goyanes A., Det-Amornrat U., Wang J., Basit A.W., Gaisford S. 3D scanning and 3D printing as innovative technologies for fabricating personalized topical drug delivery systems. J. Control. Release. 2016;234:41–48. doi: 10.1016/j.jconrel.2016.05.034. [PubMed] [CrossRef] [Google Scholar]
16. Goyanes A., Wang J., Buanz A., Martínez-Pacheco R. , Telford R., Gaisford S., Basit A.W. 3D Printing of Medicines: Engineering Novel Oral Devices with Unique Design and Drug Release Characteristics. Mol. Pharm. 2015;12:4077–4084. doi: 10.1021/acs.molpharmaceut.5b00510. [PubMed] [CrossRef] [Google Scholar]
17. Shen S., Wang H., Xue Y., Yuan L., Zhou X., Zhao Z., Dong E., Liu B., Liu W., Cromeens B., et al. Freeform fabrication of tissue-simulating phantom for potential use of surgical planning in conjoined twins separation surgery. Sci. Rep. 2017;7:11048. doi: 10.1038/s41598-017-08579-6. [PMC free article] [PubMed] [CrossRef] [Google Scholar]
18. Huang J.-J., Ren J.-A., Wang G.-F., Li Z.-A., Wu X.-W., Ren H.-J., Liu S. 3D-printed “fistula stent” designed for management of enterocutaneous fistula: An advanced strategy. World J. Gastroenterol. 2017;23:7489–7494. doi: 10.3748/wjg.v23.i41.7489. [PMC free article] [PubMed] [CrossRef] [Google Scholar]
19. Almeida C.R., Serra T., Oliveira M.I., Planell J.A., Barbosa M.A., Navarro M. Impact of 3-D printed PLA- and chitosan-based scaffolds on human monocyte/macrophage responses: Unraveling the effect of 3-D structures on inflammation. Acta Biomater. 2014;10:613–622. doi: 10.1016/j.actbio.2013.10.035. [PubMed] [CrossRef] [Google Scholar]
20. Rhee S., Puetzer J.L., Mason B.N., Reinhart-King C.A., Bonassar L.J. 3D Bioprinting of Spatially Heterogeneous Collagen Constructs for Cartilage Tissue Engineering. ACS Biomater. Sci. Eng. 2016;2:1800–1805. doi: 10.1021/acsbiomaterials.6b00288. [CrossRef] [Google Scholar]
21. Laronda M.M., Rutz A.L., Xiao S., Whelan K.A., Duncan F.E., Roth E.W., Woodruff T.K., Shah R.N. A bioprosthetic ovary created using 3D printed microporous scaffolds restores ovarian function in sterilized mice. Nat. Commun. 2017;8:15261. doi: 10.1038/ncomms15261. [PMC free article] [PubMed] [CrossRef] [Google Scholar]
22. Markstedt K., Mantas A., Tournier I., Martínez Ávila H., Hägg D., Gatenholm P. 3D Bioprinting Human Chondrocytes with Nanocellulose–Alginate Bioink for Cartilage Tissue Engineering Applications. Biomacromolecules. 2015;16:1489–1496. doi: 10.1021/acs.biomac.5b00188. [PubMed] [CrossRef] [Google Scholar]
23. Nguyen D., Hägg D.A., Forsman A., Ekholm J., Nimkingratana P., Brantsing C., Kalogeropoulos T., Zaunz S., Concaro S., Brittberg M., et al. Cartilage Tissue Engineering by the 3D Bioprinting of iPS Cells in a Nanocellulose/Alginate Bioink. Sci. Rep. 2017;7:658. doi: 10.1038/s41598-017-00690-y. [PMC free article] [PubMed] [CrossRef] [Google Scholar]
24. Tan Z., Parisi C., Di Silvio L., Dini D., Forte A.E. Cryogenic 3D Printing of Super Soft Hydrogels. Sci. Rep. 2017;7:16293. doi: 10.1038/s41598-017-16668-9. [PMC free article] [PubMed] [CrossRef] [Google Scholar]
25. Lee J.-S., Hong J.M., Jung J.W., Shim J.-H., Oh J.-H., Cho D.-W. 3D printing of composite tissue with complex shape applied to ear regeneration. Biofabrication. 2014;6:24103. doi: 10.1088/1758-5082/6/2/024103. [PubMed] [CrossRef] [Google Scholar]
26. Phillippi J.A., Miller E., Weiss L., Huard J. , Waggoner A., Campbell P. Microenvironments Engineered by Inkjet Bioprinting Spatially Direct Adult Stem Cells Toward Muscle- and Bone-Like Subpopulations. Stem Cells. 2008;26:127–134. doi: 10.1634/stemcells.2007-0520. [PubMed] [CrossRef] [Google Scholar]
27. Duan B., Hockaday L.A., Kang K.H., Butcher J.T. 3D bioprinting of heterogeneous aortic valve conduits with alginate/gelatin hydrogels. J. Biomed. Mater. Res. A. 2013;101:1255–1264. doi: 10.1002/jbm.a.34420. [PMC free article] [PubMed] [CrossRef] [Google Scholar]
28. Fedorovich N.E., Alblas J., de Wijn J.R., Hennink W.E., Verbout A.J., Dhert W.J.A. Hydrogels as Extracellular Matrices for Skeletal Tissue Engineering: State-of-the-Art and Novel Application in Organ Printing. Tissue Eng. 2007;13:1905–1925. doi: 10.1089/ten.2006.0175. [PubMed] [CrossRef] [Google Scholar]
29. Hsieh F.-Y., Lin H.-H., Hsu S. 3D bioprinting of neural stem cell-laden thermoresponsive biodegradable polyurethane hydrogel and potential in central nervous system repair. Biomaterials. 2015;71:48–57. doi: 10.1016/j.biomaterials.2015.08.028. [PubMed] [CrossRef] [Google Scholar]
30. Suntornnond R., An J., Chua C.K. Roles of support materials in 3D bioprinting. Int. J. Bioprint. 2017;3:83–89. doi: 10.18063/IJB.2017.01.006. [CrossRef] [Google Scholar]
31. Poldervaart M.T., Goversen B., de Ruijter M., Abbadessa A., Melchels F.P.W., Öner F.C., Dhert W.J.A., Vermonden T., Alblas J. 3D bioprinting of methacrylated hyaluronic acid (MeHA) hydrogel with intrinsic osteogenicity. PLoS ONE. 2017;12:e0177628. doi: 10.1371/journal.pone.0177628. [PMC free article] [PubMed] [CrossRef] [Google Scholar]
32. Sa M.-W., Nguyen B.-N.B., Moriarty R.A., Kamalitdinov T., Fisher J.P., Kim J.Y. Fabrication and evaluation of 3D printed BCP scaffolds reinforced with ZrO2 for bone tissue applications. Biotechnol. Bioeng. 2017:1–11. doi: 10.1002/bit.26514. [PMC free article] [PubMed] [CrossRef] [Google Scholar]
33. Qi X., Pei P., Zhu M., Du X., Xin C. , Zhao S., Li X., Zhu Y. Three dimensional printing of calcium sulfate and mesoporous bioactive glass scaffolds for improving bone regeneration in vitro and in vivo. Sci. Rep. 2017;7:42556. doi: 10.1038/srep42556. [PMC free article] [PubMed] [CrossRef] [Google Scholar]
34. Sun M., Liu A., Shao H., Yang X., Ma C., Yan S., Liu Y., He Y., Gou Z. Systematical Evaluation of Mechanically Strong 3D Printed Diluted magnesium Doping Wollastonite Scaffolds on Osteogenic Capacity in Rabbit Calvarial Defects. Sci. Rep. 2016;6:34029. doi: 10.1038/srep34029. [PMC free article] [PubMed] [CrossRef] [Google Scholar]
35. Wang Y., Wang K., Li X., Wei Q., Chai W., Wang S., Che Y., Lu T., Zhang B. 3D fabrication and characterization of phosphoric acid scaffold with a HA/β-TCP weight ratio of 60:40 for bone tissue engineering applications. PLoS ONE. 2017;12:e0174870. doi: 10.1371/journal.pone.0174870. [PMC free article] [PubMed] [CrossRef] [Google Scholar]
36. Sandler N., Määttänen A., Ihalainen P. , Kronberg L., Meierjohann A., Viitala T., Peltonen J. Inkjet printing of drug substances and use of porous substrates-towards individualized dosing. J. Pharm. Sci. 2011;100:3386–3395. doi: 10.1002/jps.22526. [PubMed] [CrossRef] [Google Scholar]
37. Uddin M.J., Scoutaris N., Klepetsanis P., Chowdhry B., Prausnitz M.R., Douroumis D. Inkjet printing of transdermal microneedles for the delivery of anticancer agents. Int. J. Pharm. 2015;494:593–602. doi: 10.1016/j.ijpharm.2015.01.038. [PubMed] [CrossRef] [Google Scholar]
38. Strobel L.A., Rath S.N., Maier A.K., Beier J.P., Arkudas A., Greil P., Horch R.E., Kneser U. Induction of bone formation in biphasic calcium phosphate scaffolds by bone morphogenetic protein-2 and primary osteoblasts. J. Tissue Eng. Regen. Med. 2014;8:176–185. doi: 10.1002/term.1511. [PubMed] [CrossRef] [Google Scholar]
39. Inzana J.A., Trombetta R.P., Schwarz E.M., Kates S.L., Awad H.A. 3D printed bioceramics for dual antibiotic delivery to treat implant-associated bone infection. Eur. Cells Mater. 2015;30:232–247. doi: 10.22203/eCM.v030a16. [PMC free article] [PubMed] [CrossRef] [Google Scholar]
40. Boehm R.D., Miller P.R., Daniels J., Stafslien S., Narayan R.J. Inkjet printing for pharmaceutical applications. Mater. Today. 2014;17:247–252. doi: 10.1016/j.mattod.2014.04.027. [CrossRef] [Google Scholar]
41. Asadi-Eydivand M., Solati-Hashjin M., Shafiei S.S., Mohammadi S., Hafezi M., Osman N.A.A. Structure; properties; and in vitro behavior of heat-treated calcium sulfate scaffolds fabricated by 3D printing. PLoS ONE. 2016;11:e0151216. doi: 10.1371/journal.pone.0151216. [PMC free article] [PubMed] [CrossRef] [Google Scholar]
42. Inzana J.A., Olvera D., Fuller S.M., Kelly J.P., Graeve O.A., Schwarz E.M., Kates S.L., Awad H.A. 3D printing of composite calcium phosphate and collagen scaffolds for bone regeneration. Biomaterials. 2014;35:4026–4034. doi: 10.1016/j.biomaterials.2014.01.064. [PMC free article] [PubMed] [CrossRef] [Google Scholar]
43. Farzadi A., Solati-Hashjin M., Asadi-Eydivand M., Osman N.A.A. Effect of layer thickness and printing orientation on mechanical properties and dimensional accuracy of 3D printed porous samples for bone tissue engineering. PLoS ONE. 2014;9:e108252. doi: 10.1371/journal.pone.0108252. [PMC free article] [PubMed] [CrossRef] [Google Scholar]
44. Wickström H., Hilgert E., Nyman J., Desai D., Şen Karaman D., de Beer T., Sandler N., Rosenholm J. Inkjet Printing of Drug-Loaded Mesoporous Silica Nanoparticles—A Platform for Drug Development. Molecules. 2017;22:2020. doi: 10.3390/molecules22112020. [PMC free article] [PubMed] [CrossRef] [Google Scholar]
45. Meess K.M., Izzo R.L., Dryjski M.L., Curl R.E., Harris L.M., Springer M., Siddiqui A.H., Rudin S., Ionita C.N. 3D Printed Abdominal Aortic Aneurysm Phantom for Image Guided Surgical Planning with a Patient Specific Fenestrated Endovascular Graft System. Proc. SPIE Int. Soc. Opt. Eng. 2017;10138:101380P. [PMC free article] [PubMed] [Google Scholar]
46. Kuroda S., Kobayashi T., Ohdan H. 3D printing model of the intrahepatic vessels for navigation during anatomical resection of hepatocellular carcinoma. Int. J. Surg. Case Rep. 2017;41:219–222. doi: 10.1016/j.ijscr.2017.10.015. [PMC free article] [PubMed] [CrossRef] [Google Scholar]
47. Li B., Wei H., Zeng F., Li J., Xia J.J., Wang X. Application of A Novel Three-dimensional Printing Genioplasty Template System and Its Clinical Validation: A Control Study. Sci. Rep. 2017;7:5431. doi: 10.1038/s41598-017-05417-7. [PMC free article] [PubMed] [CrossRef] [Google Scholar]
48. Gear J.I., Cummings C., Craig A.J., Divoli A., Long C.D.C., Tapner M., Flux G.D. Abdo-Man: A 3D-printed anthropomorphic phantom for validating quantitative SIRT. EJNMMI Phys. 2016;3:17. doi: 10.1186/s40658-016-0151-6. [PMC free article] [PubMed] [CrossRef] [Google Scholar]
49. Zein N.N., Hanouneh I.A., Bishop P.D., Samaan M., Eghtesad B., Quintini C., Miller C., Yerian L., Klatte R. Three-dimensional print of a liver for preoperative planning in living donor liver transplantation. Liver Transplant. 2013;19:1304–1310. doi: 10.1002/lt.23729. [PubMed] [CrossRef] [Google Scholar]
50. Waran V., Narayanan V., Karuppiah R., Owen S.L.F., Aziz T. Utility of multimaterial 3D printers in creating models with pathological entities to enhance the training experience of neurosurgeons. J. Neurosurg. 2014;120:489–492. doi: 10.3171/2013.11.JNS131066. [PubMed] [CrossRef] [Google Scholar]
51. Mitsouras D., Lee T.C., Liacouras P., Ionita C.N., Pietilla T., Maier S.E., Mulkern R.V. Three-dimensional printing of MRI-visible phantoms and MR image-guided therapy simulation. Magn. Reson. Med. 2017;77:613–622. doi: 10.1002/mrm.26136. [PMC free article] [PubMed] [CrossRef] [Google Scholar]
52. Lan Q., Chen A., Zhang T., Li G., Zhu Q., Fan X., Ma C., Xu T. Development of Three-Dimensional Printed Craniocerebral Models for Simulated Neurosurgery. World Neurosurg. 2016;91:434–442. doi: 10.1016/j.wneu.2016.04.069. [PubMed] [CrossRef] [Google Scholar]
53. Sander I., Liepert T. , Doney E., Leevy W., Liepert D. Patient Education for Endoscopic Sinus Surgery: Preliminary Experience Using 3D-Printed Clinical Imaging Data. J. Funct. Biomater. 2017;8:13. doi: 10.3390/jfb8020013. [PMC free article] [PubMed] [CrossRef] [Google Scholar]
54. Dwivedi D.K., Chatzinoff Y., Zhang Y., Yuan Q., Fulkerson M., Chopra R., Brugarolas J., Cadeddu J.A., Kapur P., Pedrosa I. Development of a Patient-specific Tumor Mold Using Magnetic Resonance Imaging and 3-Dimensional Printing Technology for Targeted Tissue Procurement and Radiomics Analysis of Renal Masses. Urology. 2017 doi: 10.1016/j.urology.2017.08.056. in press. [PMC free article] [PubMed] [CrossRef] [Google Scholar]
55. Miller J.S., Stevens K.R., Yang M.T., Baker B.M., Nguyen D.-H.T., Cohen D.M., Toro E., Chen A.A., Galie P.A., Yu X., et al. Rapid casting of patterned vascular networks for perfusable engineered three-dimensional tissues. Nat. Mater. 2012;11:768–774. doi: 10.1038/nmat3357. [PMC free article] [PubMed] [CrossRef] [Google Scholar]
56. Kolesky D.B., Truby R.L., Gladman A.S., Busbee T.A., Homan K.A., Lewis J.A. 3D bioprinting of vascularized; heterogeneous cell-laden tissue constructs. Adv. Mater. 2014;26:3124–3130. doi: 10.1002/adma.201305506. [PubMed] [CrossRef] [Google Scholar]
57. Hinton T.J., Jallerat Q., Palchesko R.N., Park J.H., Grodzicki M.S., Shue H.-J., Ramadan M.H., Hudson A.R., Feinberg A.W. Three-dimensional printing of complex biological structures by freeform reversible embedding of suspended hydrogels. Sci. Adv. 2015;1:e1500758. doi: 10.1126/sciadv.1500758. [PMC free article] [PubMed] [CrossRef] [Google Scholar]
58. Hassana B.O., Guessasma S., Belhabib S., Nouri H. Explaining the Difference between Real Part and Virtual Design of 3D Printed Porous Polymer at the Microstructural Level. Macromol. Mater. Eng. 2016;301:566–576. doi: 10.1002/mame.201500360. [CrossRef] [Google Scholar]
59. Ventola C.L. Medical Applications for 3D Printing: Current and Projected Uses. Pharm. Ther. 2014;39:704–711. [PMC free article] [PubMed] [Google Scholar]
all about printing organs on a 3D printer
Printing organs on a 3D printer or bioprinting is a promising technology for growing healthy and living organs to replace damaged or missing ones. In addition to a 3D printer, bioprinting requires a model of an organ, patient cell material, and an environment where the organ will remain until implantation.
Printed organs are better than prostheses and transplanted body parts. Their capabilities are identical to native ones and they are not rejected by the immune system if they are created from the patient's DNA. Bioprinting will reduce the time to obtain the desired organ and save the lives of patients who need an immediate transplant. nine0005
Printing organs on a 3D printer has already been successfully tested on animals. Scientists at Northwestern University implanted artificial ovaries in sterilized mice and they gave birth to healthy mice. In the Chinese company Sichuan Revotek, rhesus monkeys have been implanted with blood vessels grown from the material of the same monkeys.
From human body parts, only internal tissues and skin are printed so far. Reduced but working copies of ears and noses are created. The first printing of human organs is expected by 2030. nine0005
How bioprinting works
Research groups or companies are developing different bioprinting concepts:
- Wireframe. The growth of living cells on an inorganic base, which disappears with the development of natural connections between cells. The main difficulty is to find a material that is as elastic or rigid as the organ being replaced. It must degrade quickly so as not to interfere with the strengthening of the extracellular matrix and dissolve without leaving toxic compounds. Hydrogel, titanium, gelatin, synthetic and biopolymers are suitable for wireframe printing. nine0020
- Frameless. Application of prepared cells on a hydrogel base. While the cells are in the printer, they are cooled and are in thin hydrogel spheroids.
When printing, the temperature rises to 36.6°C, the spheroids scatter, and the cells gradually form their own natural framework - the cellular matrix. This printing is less common than wireframe printing - it appeared later and is more difficult to reproduce.
- Mimicry. The technology of the future, involves the creation of complete copies of organs at once. For it, bioprinting is being developed at the molecular level and in-depth studies of the nature of cells are being carried out. nine0021
Methods for 3D printing of organs
Inkjet. The first devices for bioprinting were inkjet, conventional printers also use this method. They store biological material in cartridges that are sprayed onto a hydrogel substrate like paint on paper. Disadvantages - inaccurate droplet ejection and blockage of the spray nozzle with possible death of cellular material. Inkjet printing of organs on a printer is not suitable for viscous materials because they are not atomized. The scope is limited to the restoration of bone, cartilage, muscles and skin. Advantages - low cost and mass reproducibility. nine0005
Microextrusion. This method is used in inorganic 3D printing. For printing, a pneumatic supply of material is used in a movable extruder head, which stacks the cells. The more heads, the more accurate and faster the printer. Disadvantages - the denser the cells fit, the less they survive. With a comparable stacking density, more cells die from microextrusion printing than from inkjet printing. Advantages - suitable for 3D printing of high-density organs, fine-tuning of the material supply due to pressure regulation. nine0005
Laser. Common in industry but used in bioprinting. A laser is used to heat glass with a liquid cell substrate. At the beam concentration point, excess pressure is created, which pushes the cells to the desired area of the substrate. A reflective element is placed between the beam and the glass with biomaterial, which reduces the power of the beam. Disadvantages - increased metal content in the cells from the evaporation of the reflective element. Price. Advantages - controlled up to individual cells, laying of biomaterial. nine0005
Who offers 3D printing of organs
Bioprinting companies that offer 3D printing of organs or sell bioprinters:
- Organovo - San Diego, USA. Prints and sells liver tissue " exVive3D" to pharmaceutical companies. In 2009, Organovo, together with the Austrian Invetech, launched the first mass-produced bioprinter, Novogen.
- BioBots is a startup that presented a cheap commercial bioprinter at TechCrunch 2013. Today, the Biobot 1 model is available for purchase, Biobot 2 is still in development, but already presented on the company's website. nine0021
- 3D Bioprinting Solutions - Russia , Moscow. Focused on frameless printing, has developed its FABION 3D printer and is working on its own organoprinting technology
- Cyfuse Biomedical - Tokyo, Japan.
They developed the Regenovo bioprinter, which was used to print skin and successfully grew 2-mm vessels.
How much does a 3D bioprinter cost
The average cost of a bioprinter is a quarter of a million dollars, but budget models are available for up to $10,000. Most printers available for purchase are extrusion type and work with frame printing. nine0005
- 3D Bioplotter - $200,000 Envision TEC, Germany.
- Novogen MMX - $250.00. Organovo, USA.
- Biobot 1 - $10,000. Biobots, USA.
- 3DDiscovery - $200,000. RegenHU & Biofactory, Switzerland.
- BioAssemblyBot - $160,000 Advanced Solutions, The Netherlands.
Supporting a patient with life support devices costs about $75,000 per year. In 10 years, the patient will spend $1 million. The printer costs $200,000 and the operation costs about the same. Considering how much it costs to print organs, the operation using 3D bioprinting is reduced by 50%. nine0005
The Future of Bioprinting
3D bioprinting has gone from concept to working and commercially successful technology. So far, the main clients of bioprinting companies are large pharmaceutical corporations. They speed up drug testing by testing them directly on printed human tissues.
Expensive bioprinters won't be in city clinics in 5 years, but some patients are already recovering thanks to 3D printing. The jaw of an 83-year-old woman from Belgium was struck by osteomyelitis. The restoration was more expensive and would have taken longer than the removal of the diseased jaw and the implantation of a printed new one. A team of doctors led by Professor Jules Poukan performed the operation and the woman was able to speak immediately after the operation. The development of bioprinting will lead to medical practices where it is easier to remove an injured limb and grow a new one than to treat injuries that are now treated without amputation. nine0005
Medicine of the distant future minimizes mechanical intervention in the body. The scalpel will remain in the past - a swarm of nanorobots will print organs immediately inside the body. In 2018, a full-fledged printing of a human organ on a printer is planned - the kidneys. Then the bronchi, arteries and heart will be printed. But even clinical trials on humans are about 10 years away, and mass 3D printing of human organs and body parts will come even later.
In addition to doctors, bioprinting is attractive to cosmetologists and plastic surgeons. The desire to remain young and beautiful, and not the treatment of rare and complex diseases, will make 3D printing of human organs mass. Perhaps the people of the future will change organs and appearance as easily as smartphones. nine0005
Bioprinter: 3D printing of organs
3D bioprinting is an advanced technology that saves lives and helps create new medicines. What is the peculiarity and complexity of developments, who is engaged in them and what successes have already been made - this will be discussed in the material. We will also get acquainted with all the methods of bioprinting known today.
- How bioprinters print and what is 3D bioprinting
- The first biological 3D printer
- 3D bioprinting technologies
- Multimaterial Multinozzle 3D (MM3D) Technology, Wyss Institute
- Sound-Induced Morphogenesis (SIM) Technology, mimiX Biotherapeutics
- Biopixlar Bioprinter, Fluicell
- CELLINK Bioprinters
- Bioprinter that creates micro-human ears for patients University of Wollongong
- Bioink
- 3D Bioprinting Solutions
- What do 3D bioprinters print?
- Where do they print? nine0021
- 3D bioprinters in Russia
How bioprinters are printed and what is 3D bioprinting
3D bioprinting is the creation of 3D models using biomaterial, which includes living cells. It is used to reproduce complex structures such as skin tissue or blood vessels.
Model cells are taken from the patient and cultured until their mass is sufficient to create a bioink.
The resulting ink is loaded into the printer, which prints the desired model. nine0005
Getting enough cells is not always possible, so seaweed or porcine collagen protein comes to the rescue. Stem cells are also used, which have the ability to become any cell in the body.
The first biological 3D printer
The first mass-produced bioprinter was released by the American company Organovo by the end of 2009. The Australian company Invetech became its industrial partner. Thanks to joint efforts, the machine was born, which in 2010 printed the first full-fledged blood vessel. nine0005
Representatives of Organovo decided to move away from the idea of growing organs in a test tube and suggested that it would be much more efficient to print it. They came up with the NovoGen technology, which regulated all the interactions between the biological component of the process and its mechanical part. Invetech was involved to implement the idea. The collaboration resulted in a compact device with an intuitive interface.
The printer had two printheads. One was filled with the necessary biomaterial, the second - with auxiliary components (collagen supporting the hydrogel, growth factors). Printing accuracy reached micrometers, which played an important role in the correct placement of cells. nine0005
3D bioprinting technologies
There are several technologies and approaches being developed and applied in the field of bioprinting. Scientists and researchers from private companies and institutions work on each of them.
Technology Multimaterial Multinozzle 3D (MM3D), Wyss Institute
The technology developed at the institute is based on the use of fast moving high pressure valves. The applied method makes it possible to switch between materials up to 50 times per second. This speed is faster than you can see with the naked eye. nine0005
The printheads themselves are 3D printed, so they can be easily customized to your specific needs.
The technology is suitable for the manufacture of complex objects, including moving robots. The method significantly speeds up the creation of complex models, because the printheads can use several nozzles at once.
Sound-Induced Morphogenesis (SIM) Technology by mimiX Biotherapeutics
Swiss technology is based on the reproduction of well-defined biological patterns that self-assemble into functional tissues using sound waves. The method embodies a highly efficient sequence for reproducing organized and dense cellular structures. nine0005
The mimiX technology uses sound waves. The space for growing cells is formed around a given type of speaker. Depending on the shape of the cup and the sound produced, structures of various shapes, such as gratings, are formed.
The creation of this method serves an important purpose - the availability and speed of playback, which can be achieved in any room. Prior to the invention of the SIM, bioprinting was available for scientific research. For clinical, it has become too complicated and lengthy. Now this has changed. nine0005
Fluicell Biopixlar Bioprinter
Swedish-based Fluicell focuses on creating platforms for studying cell behavior. Her method of work makes it possible to make complex structures that mimic tissue, in which the location of individual cells is controlled by a gamepad. The workflow is similar to a video game.
The company uses the micro-jet technique. Thanks to the micro jet tube and the precision of the pump during the direction of the biomaterial to the printing zone, it gives micro-level control over the material. Because of this, systems scale to the macro level naturally. High resolution prints are produced. nine0005
The method makes it possible to reproduce multicomponent structures, while the material can be created in the printer itself. This approach eliminates the need for laboratory preparation. The mixing progress of different materials is controlled in the microfluidic chamber. The result is a 3D printed finished structure, which was created without the use of gels and scaffolds.
The technical capabilities of this method make it ideal for processing scarce materials such as biopsy specimens, stem cells and primary cells. nine0005
CELLINK Bioprinters
CELLINK is a Swedish company that develops bioprinting technologies for applications in various fields, including cosmetics and medicine. The principles generated by the organization work to create skin tissue, cartilage, liver and other products.
In 2019, the manufacturer launched two models of bioprinters on the market - Bio X6 and Lumen X. The first one is designed for creating constructs with any type of cells. With it, you can reproduce any tissue found in the body. The company focuses on quick results and combining a large number of materials. nine0005
The Bio X6 has 6 printheads built in with CELLINK Clean Camera Technology. It is equipped with a smart interchangeable head method and two powerful fans to create excess air pressure inside the chamber. The user gets the opportunity to combine several materials in one print with a structure of increased complexity.
The second Lumen X unit is the result of a collaboration with the American company Volumetric. This is a startup focused on creating bioprinters using SLA technology. The device has a low cost and modest dimensions. At the same time, it has high printing accuracy and excellent performance. Such characteristics are especially important for the creation of vascular structures. Lumen X gets the job done 10 times faster than its competitors under the same conditions. nine0005
Bioprinter for Microtia Patients Creating Human Ears, University of Wollongong
Myctoria is a congenital defect associated with developmental arrest of the outer ear. It is found during pregnancy. Since the structure of the ear has a specific shape, the treatment of its deformity causes serious difficulties. According to the creators of the bioprinter, the new technology is leading to a revolution in helping children with microtia.
The Australian University of Wollongong created the Alek 3D printer. It prints human ears for further use in reconstructive surgery. Stem cells serve as the basis for bioink. The creation of ears on a bioprinter looks very promising, since the method allows you to design a transplant according to the shape of the patient's face and do it in a fairly short time. The technology excludes the search for donors to take a piece of cartilage, the work is based on the use of the patient's natural tissues. nine0005
Bioink
Manufacturers are developing not only the technology and design of printing machines, but also materials that can be used to create complex structures and entire organs.
Allevi Liver Tissue Ink
The complexity of reproducing the liver lies in the fact that it has many important functions for life. There are more than five hundred of them. A small number of manufacturers are able to create bio-ink for the liver that will meet all the necessary conditions. nine0005
The American company Allevi is one such company. The drug, which makes it possible to reproduce tissue-like structures that mimic the natural characteristics of tissues, can be freely purchased through the Allevi online store.
Biogelx Synthetic Bioink
Biogelx is a Scottish company. It is based in a laboratory at the University of Strathclyde in Glasgow. The direction of the company is the research and creation of artificial materials for bioprinting.
Hydrogel ink has a unique chemical and physical variability. It allows you to accurately recreate a variety of tissue parameters. As a result, cells are able to interact in an almost natural environment.
Ink helps keep cells alive. They provide a simple crosslinking technique and viscosity control as well as a high degree of reproducibility. Due to its positive technical abilities, the material is compatible with a wide range of 3D bioprinters. nine0005
Hydrogel with mineral nanoparticles, University of Texas
American development from the staff of the University of Texas (TAMU). Scientists have created a material in the form of a hydrogel. It contains mineral nanoparticles. They have the ability to carry out protein preparations to control the behavior of cells. Inks of this kind help in the field of creating tissues containing blood vessels.
Developers have been developing hydrogel bioinks based on the inert polymer PEG (polyethylene glycol). Printing with this type of ink is difficult due to its low viscosity. In the course of research, it turned out that silicate nanoparticles effectively increase the degree of viscosity, while almost not changing the other technical parameters of the printed material. Thus, TAMU workers have created a new class of hydrogels. nine0005
Bio-ink for artificial leather printing, Renseller Polytechnic Institute
The production of high-quality skin imitations after burns and other wounds has occupied the minds of bioengineers for many years. There are 2 ways to treat serious skin lesions. The first is the use of autologous skin grafts. Healthy tissue is taken from the patient and transplanted to damaged areas, while fresh wounds are formed and, in general, the procedure is quite unpleasant and painful. nine0005
The second method is to use skin substitutes made from foreign materials, such as bovine collagen. Such imitations do not completely cover deep wounds and are very different from natural skin.
Skin resurfacing technology from a collaboration between Rensselaer Polytechnic Institute (RPI) and Yale University promises to revolutionize skin grafting. They created bio-ink using living human cells. The material helps in reproducing artificial skin, which later itself recreates the system of blood vessels. nine0005
3D Bioprinting Solutions
3D Bioprinting Solutions is the only organization in Russia dealing with bioprinting. The company was founded in 2013. One of the co-founders was the co-founder of INVITRO - Alexander Ostrovsky. His specialty is a resuscitator.
In 2014, the laboratory presented the first bioprinter made in Russia. He received the name FABION. According to the list of the use of various printed materials, this device is one of the leaders in the field of multifunctional devices. nine0005
In early 2015, the company created and successfully transplanted a mouse thyroid organ construct. In 2016, researchers produced a printhead that can automatically feed tissue spheroids for 3D bioprinting. The development was applied in the new version of the device - FABION-2.
After the release of an updated version of the device, the company's specialists focused on creating a printer that uses a new working methodology, different from previous solutions. It was the principle of magnetic levitation and the ability of microtissues to self-assemble from tissue spheroids. A fully functioning magnetic assembly saw the light of day by the spring of 2017. nine0005
At the end of the summer of 2017, cooperation with the state company Roscosmos began. The laboratory has signed a contract for the implementation of a space biofabrication experiment aboard the Russian part of the ISS. Scientists have begun to develop the Organ.Avt printer capable of working in zero gravity.
The Magnetic Bioprinter experiment started at the end of 2018. In its course, models of human bone and cartilage tissue, as well as the mouse thyroid gland, were printed. To carry out the work, the Soyuz MS-11 crew members underwent appropriate training at the 3D Bioprinting Solutions laboratory. nine0005
In addition to bioprinters, the company has developed a line of multifunctional collagen products - Wiscoll. It is suitable for use in any 3D bioprinter. Bioink is used for a wide range of biofabrication experiments. The product is a concentrated solution of collagen of the first type of the highest degree of purification. It is immediately ready for use.
What is printed on 3D bioprinters?
Printing organs
Printing fully functional complex internal organs is not yet possible, although research is ongoing in this area. For example, the bladder has already managed to reproduce. It happened in 2013 in the USA (Wake Forest University). nine0005
Scientists have extracted raw material from a patient's poorly functioning organ, nurtured them and added nutrients. Next, they reproduced the shape of the bladder according to the parameters of the patient and cultured cells impregnated through it. The model was placed in an incubator, brought to the required condition and transplanted into a human. Over time, it collapsed, leaving completely organic material in its place.
The same team produced viable urethras. Research is ongoing and breakthroughs are being made in the creation of the kidneys, liver and heart. nine0005
Printing of tissues and vessels
The human body is pierced by tens of thousands of kilometers of capillaries, arteries and veins. Over time, they wear out, and scientists are conducting research on the possibility of their full replacement. Creating body parts on a bioprinter is impossible without reproducing viable blood vessels. Technologies make it possible to create materials designed for further favorable independent development of blood vessels.
Surgical practice
Surgeons got the opportunity to practice on operations on organs and tissues that look 100% real. This happens both with the help of virtual prototyping and with the use of 3D printed models.
New drug testing
Bioprinted tissue has several cell types with different densities and key architectural features. This makes it possible to conduct research on the impact of diseases on the body, as well as to work out various methods of treatment. nine0005
Where do they print?
Organizations that offer organ printing or sell bioprinters:
- 3D Bioprinting Solutions - Russia, Moscow. Specializes in frameless printing, created two printers - FABION and FABION-2. Develops his own method of organoprinting.
- Organovo - USA, San Diego. Produces and sells liver tissue to pharmaceutical companies.
In 2009, the first mass-produced bioprinter, Novogen, was released.
- BioBots - USA, Louisville. A startup that introduced a cheap bioprinter for commercial use in 2013. Available BioBot BASIC. Work is underway on the second version of the device. nine0021
- Cyfuse Biomedical - Japan, Tokyo. The company produced the Regenovo bioprinter, which can be used to create skin tissues and grow blood vessels.
3D bioprinters in Russia
So far, bioprinting devices in Russia are represented by only one company created by the co-founder of the INVITRO network - 3D Bioprinting Solutions. Ongoing research on the Russian part of MSCs under weightless conditions gives researchers hope that unique data will be obtained, on the basis of which new drugs will be developed. nine0005
Scientists are also optimistic about the creation of functional complex organs of the human body, saying that they will appear already in the current century.
Emerging technologies in the field of bioprinting make it possible to draw the following conclusions:
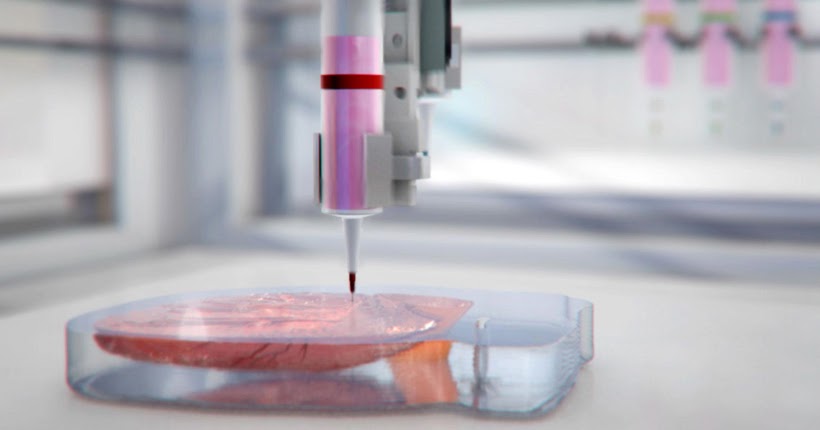